Book contents
- Extinctions
- Reviews
- Extinctions
- Copyright page
- Epigraph
- Dedication
- Contents
- Preface
- Acknowledgements
- Further Reading
- Introduction
- 1 The Anthropocene and the Earth System
- 2 A Short Detour: The Fossil Record and the Geological Time Scale
- 3 The Origin of Animals and the Emergence of the Earth System
- 4 Documenting Ancient Biodiversity
- 5 Mass Extinctions: The Basics
- 6 Causes of the End-Permian and End-Cretaceous Extinction Events
- 7 Time Heals All: Recovering from a Mass Extinction
- 8 The Late Quaternary Megafaunal Extinctions
- 9 Surviving the Anthropocene
- Book part
- Further Reading
- Index
- References
Further Reading
Published online by Cambridge University Press: 01 September 2021
- Extinctions
- Reviews
- Extinctions
- Copyright page
- Epigraph
- Dedication
- Contents
- Preface
- Acknowledgements
- Further Reading
- Introduction
- 1 The Anthropocene and the Earth System
- 2 A Short Detour: The Fossil Record and the Geological Time Scale
- 3 The Origin of Animals and the Emergence of the Earth System
- 4 Documenting Ancient Biodiversity
- 5 Mass Extinctions: The Basics
- 6 Causes of the End-Permian and End-Cretaceous Extinction Events
- 7 Time Heals All: Recovering from a Mass Extinction
- 8 The Late Quaternary Megafaunal Extinctions
- 9 Surviving the Anthropocene
- Book part
- Further Reading
- Index
- References
Summary
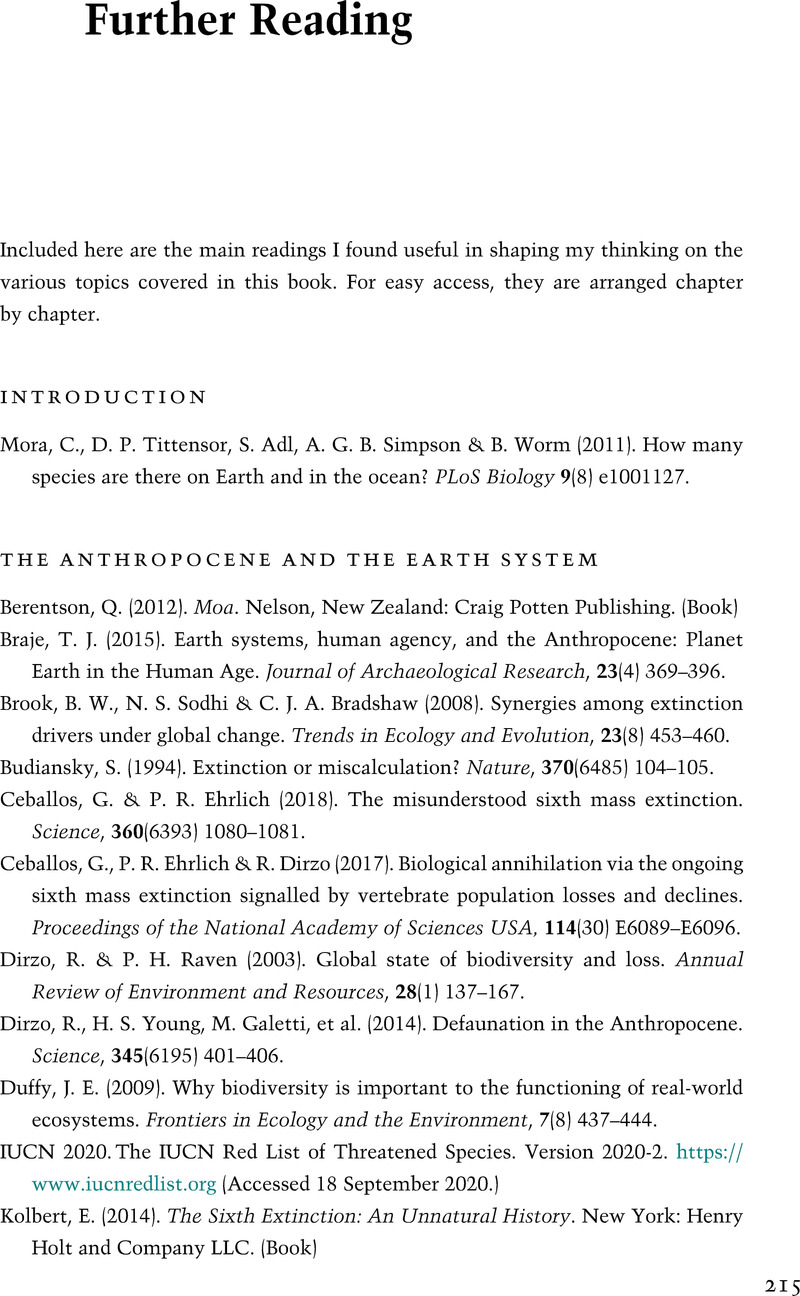
- Type
- Chapter
- Information
- ExtinctionsLiving and Dying in the Margin of Error, pp. 215 - 236Publisher: Cambridge University PressPrint publication year: 2021