Introduction
Metal additive manufacturing (AM) is no longer solely a prototyping technology; it is now being used for production of industrial parts ranging from orthopedic implants to aircraft components. Reference Isaza and Aumund-Kopp1 The last two decades have seen the development of a diversity of metal AM processes. Figure 1 summarizes these processes categorized in terms of energy source, feedstock form, and additive method with corresponding key equipment manufacturers. In principle, all weldable metals can be fusion-printed today. In a broader sense, all powdered metals can be printed at room temperature using the ExOne binder jetting process (see Figure 1) Reference Ek2 to achieve a preliminary shape, with the final shape and required properties developed through a subsequent infiltration or sintering process.
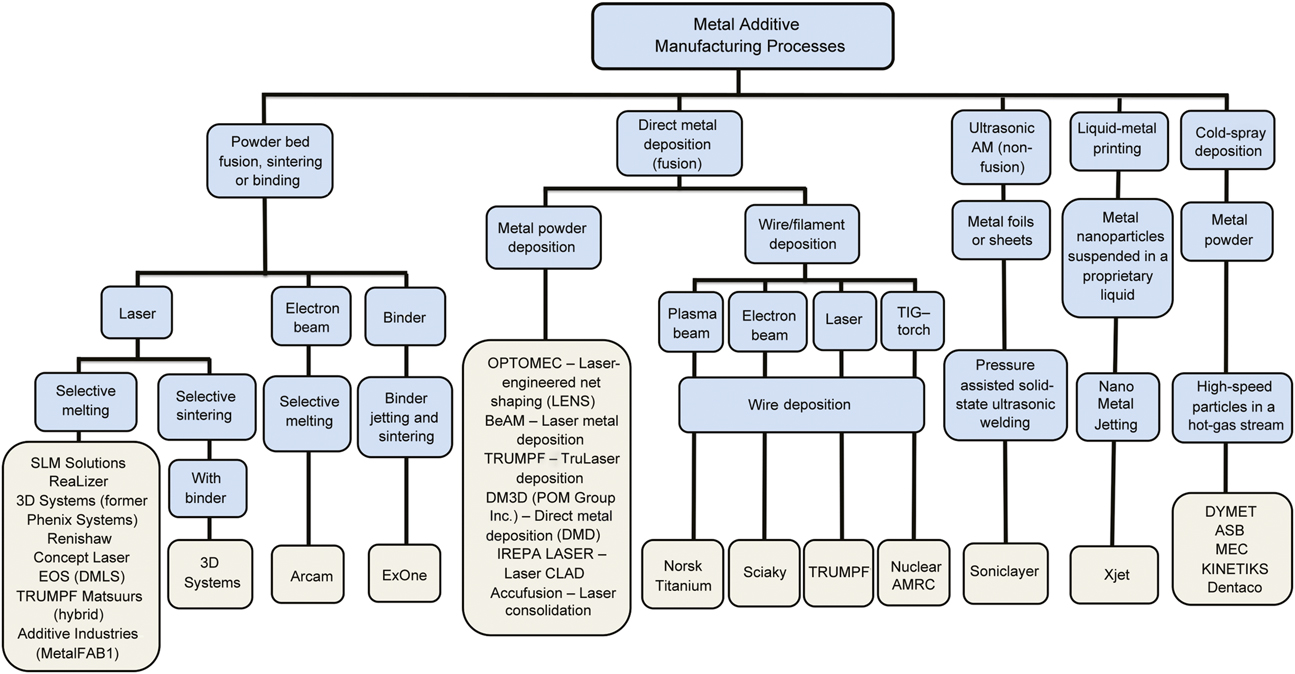
Figure 1. A schematic summary of current metal additive manufacturing (AM) processes. Reference Ek2
Titanium alloys are advanced structural materials that possess an array of unique properties. These include corrosion immunity to seawater, high strength-to-weight ratios, excellent fracture toughness, high-fatigue performance, significant compatibility with composites, long durability with little or no maintenance, and superb biocompatibility. However, titanium-based materials are not easy to machine due to their low thermal conductivity and acute reactivity. Reference Qian3 In addition, they are difficult to cast since molten titanium is extremely reactive, which is problematic for both melt handling and casting. Reference Qian3 As a result, historically, titanium components have been mostly machined from forged titanium blanks at a slow speed, entailing prolonged manufacturing cycles and up to 95% of the raw material being lost as scrap (recycling titanium chips from machining is not straightforward). Reference Barnes, Peter and Blue4,Reference Tirelli, Chiappini, Strano, Monno and Semeraro5 The concept of metal AM was therefore especially attractive for the manufacture of titanium components.
From a research perspective, Ti-6Al-4V, which accounts for more than 50% of total titanium usage, 6 has been the single most extensively studied alloy for AM. Apart from the liquid metal printing process, Ti-6Al-4V has been additively manufactured using every other AM process (shown in Figure 1) to gauge the capability of each process.
This article offers an overview of AM and postprocessing of AM Ti-6Al-4V by focusing on microstructures and mechanical properties. The discussion is limited to selective laser melting (SLM), selective electron-beam melting (SEBM), and laser metal (powder and wire) deposition (LMD).
Macro- and microstructural characteristics of AM Ti-6Al-4V
The manufacturing of each layer of a Ti-6Al-4V component by SLM, SEBM, and LMD involves small-volume melting of the feedstock material (powder or wire), near rapid solidification, and thermal influence from the subsequently built layers (excluding the last few layers).
Melting is the first major phase-transformation process involved. The liquidus temperature (T l) of Ti-6Al-4V is ∼1650 ± 15°C and its solidus temperature (T s) is ∼1605 ± 10°C. Reference Welsch, Boyer and Collings7 It should be noted that compositions of Al and V in Ti-6Al-4V are allowed to vary from 5.5–6.75 and 3.5–4.5, respectively. These variations lead to different liquidus, solidus, and density (4.42–4.43 g cm–3) values. The maximum metal pool temperature during AM of Ti-6Al-4V by laser powder deposition falls in the range of ∼2000–2500°C at a laser power of 350 W, deposition rate of 0.13 g/s, and layer thickness of 508 μm. Reference Marshall, Young, Thompson, Shamsaei, Daniewicz and Shao8 For comparison, a peak melt temperature of 2026°C was measured for laser powder deposition of H13 tool steel under similar AM conditions. Reference Craig, Wakeman, Grylls, Bullen, Thomas, Yurko and Zhang9 This high melt pool temperature can lead to a loss of both Al and V by volatilization. Reference Tang, Qian, Liu, Zhang and Yang10
The increase in oxygen in as-built Ti-6Al-4V by laser AM under argon was found to be less than 300 ppm compared to the feedstock oxygen level. Reference Qiu, Ravi, Dance, Ranson, Dilworth and Attallah11,Reference Brandl, Palm, Michailov, Viehweger and Leyens12 For AM of Ti-6Al-4V by SEBM, the oxygen content of the Ti-6Al-4V powder increased progressively from 0.08% (virgin) to 0.19% after being reused 21 times. Reference Tang, Qian, Liu, Zhang and Yang10 The critical level of oxygen for press-and-sinter Ti-6Al-4V is about 0.32%, beyond which tensile ductility drops dramatically due to several oxygen-induced microstructural changes. Reference Yan, Dargusch, Ebel and Qian13
The maximum cooling rate in the melt pool of Ti-6Al-4V varies in the approximate range of (1.2–4.0) × 104°C/s for laser powder deposition Reference Marshall, Young, Thompson, Shamsaei, Daniewicz and Shao8 (which is ∼1.83 × 104°C/s for laser powder deposition of H13 steel under similar AM conditions). Reference Craig, Wakeman, Grylls, Bullen, Thomas, Yurko and Zhang9 Molten Ti-6Al-4V solidifies as columnar prior-β grains in all fusion-based metal AM processes reported in literature. The subsequent cooling process is affected by both the number of layers and geometry of the part, and determines the pathways to microstructural development from sub-solidus temperatures to room temperature. Specifically, the pathways are dictated by (1) the initial cooling rate in the as-solidified alloy and (2) the continuously changing thermal gradient in the region of interest during the subsequent AM process.
The initial cooling rates in as-deposited Ti-6Al-4V near the melt pool during laser powder deposition Reference Marshall, Young, Thompson, Shamsaei, Daniewicz and Shao8 were found to be markedly higher than the critical cooling rates (>18–23°C Reference Dabrowski14,Reference Sieniawski, Ziaja, Kubiak, Motyka, Sieniawski and Ziaja15 or >420°C Reference Ahmed and Rack16 ) required for martensitic transformation in Ti-6Al-4V. Martensite (α′) is thus commonly observed in AM Ti-6Al-4V by SLM, SEBM, and LMD. However, it can decompose into fully lamellar α/β structures during AM. Reference Xu, Brandt, Sun, Elambasseril, Liu, Latham, Xia and Qian17,Reference Ahsan, Pinkerton, Moat and Shackleton18 Columnar prior-β grains, which are parallel to the AM build direction and show a 〈001〉β orientation, are predominant in each AM process. The 〈001〉 direction is known to be the favored growth direction of the parent β phase during solidification, which tends to be aligned with the direction of the maximum thermal gradient (i.e., the AM build direction). Reference de Formanoir, Michotte, Rigo, Germain and Godet19 Much effort has been expended to enable a successful columnar-to-equiaxed transition (CET). However, the large thermal gradient in the AM melt pool prevents the CET from developing in most cases. It was not until recently that researchers reported the CET in laser powder deposition of Ti-6Al-4V at a laser power of 1 kW, Reference Marshall, Young, Thompson, Shamsaei, Daniewicz and Shao8 informed by the CET map proposed by Kobryn and Semiatin Reference Kobryn and Semiatin20 for AM of Ti-6Al-4V. The use of high-power laser systems (e.g., 8 kW CO2 laser) makes it easier to realize CET due to the reduced thermal gradient in the melt pool. Reference Yuanhong, Hua, Jing and Weidong21 Other approaches are being developed to enable the achievement of fine equiaxed grains in AM Ti-6Al-4V. For example, applying deformation to each layer of wire-deposited Ti-6Al-4V has proved to be effective due to subsequent recrystallization. Reference Martina, Colegrove, Williams and Meyer22
SLM, SEBM, and LMD can result in similar or distinct microstructures depending on processing and feedstock conditions. Figure 2 shows some of the microstructural features observed, including martensite (α′), massive α phase (αm), lamellar α/β, and non-lamellar α and β. Reference Qiu, Ravi, Dance, Ranson, Dilworth and Attallah11,Reference Brandl, Palm, Michailov, Viehweger and Leyens12,Reference Xu, Brandt, Sun, Elambasseril, Liu, Latham, Xia and Qian17,Reference Lu, Tang, Ning, Liu, St. John and Qian23,Reference Lu, Qian, Tang, Yan, Wang and St. John24 Literature data on the critical cooling rates for β → α′, β → αm and β → α transformations differ widely. Reference Dabrowski14–Reference Ahmed and Rack16 These discrepancies can be partially attributed to the different compositions of Ti-6Al-4V used. Reference Tirelli, Chiappini, Strano, Monno and Semeraro5
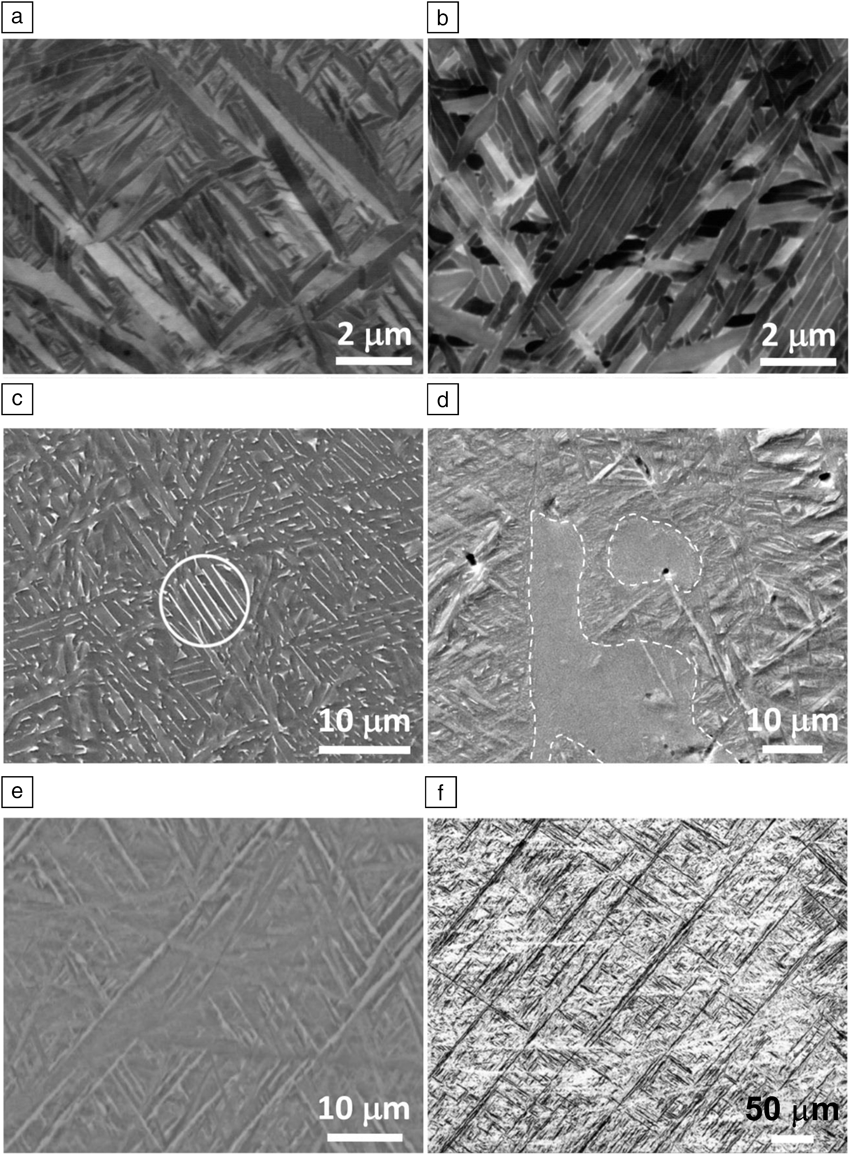
Figure 2. Microstructures of Ti-6Al-4V additively manufactured by selective laser melting (SLM), selective electron-beam melting (SEBM), and laser metal deposition. (a) Fully martensitic (α′) (SLM). Reference Xu, Brandt, Sun, Elambasseril, Liu, Latham, Xia and Qian17 (b) Fully lamellar α/β (SLM). Reference Xu, Brandt, Sun, Elambasseril, Liu, Latham, Xia and Qian17 (c) Lamellar α/β (circled area), α′ and nonlamellar α and β (SEBM), Reference Lu, Tang, Ning, Liu, St. John and Qian23 (d) α′ + massive α grains (αm) (marked areas) + lamellar α/β (SEBM). Reference Lu, Qian, Tang, Yan, Wang and St. John24 (e) α′+ partially decomposed α′ (laser powder deposition). Adapted with permission from Reference Reference Qiu, Ravi, Dance, Ranson, Dilworth and Attallah11. © 2015 Elsevier. (f) α′ + partially decomposed α′ (laser wire deposition). Adapted with permission from Reference Reference Brandl, Palm, Michailov, Viehweger and Leyens12. © 2011 Elsevier.
The borderline cooling rate for the diffusion-controlled β → α transformation is below about 20°C/s. Reference Dabrowski14–Reference Ahmed and Rack16 Above 20°C/s, either the composition-invariant β → αm massive transformation occurs, where diffusional activity occurs mainly at the advancing interfaces, Reference Massalski25 or the composition-invariant diffusionless β → α′ martensitic transformation occurs. The β → αm transformation has been identified to occur in SEBM Ti-6Al-4V, Reference Lu, Qian, Tang, Yan, Wang and St. John24 which contributes to development of SEBM Ti-6Al-4V microstructures. It should be noted that although the columnar prior-β grains show a strong 〈001〉β orientation or texture parallel to the AM build direction, the α phase that forms during subsequent cooling shows no clear texture or just a random texture. Reference Sridharan, Chaudhary, Nandwana and Babu26
Defects in AM Ti-6Al-4V
The most common defects observed in AM Ti-6Al-4V are gas-entrapped pores ( Figure 3a) Reference Lu, Tang, Ning, Liu, St. John and Qian23 and lack-of-fusion imperfections (Figure 3b), Reference Lu, Tang, Ning, Liu, St. John and Qian23 including unmelted or incompletely melted Ti-6Al-4V particles (Figure 3c). Reference Galarraga, Lados, Dehoff, Kirka and Nandwana27 The lack-of-fusion voids are also referred to as lack-of-fusion pores by some researchers. They are mostly irregular in shape and larger than gas-entrapped spherical pores. As such, they can be much more damaging than gas-entrapped spherical pores, Reference Cunningham, Narra, Ozturk, Beuth and Rollett28 especially when their orientations are perpendicular to the tensile loading direction.
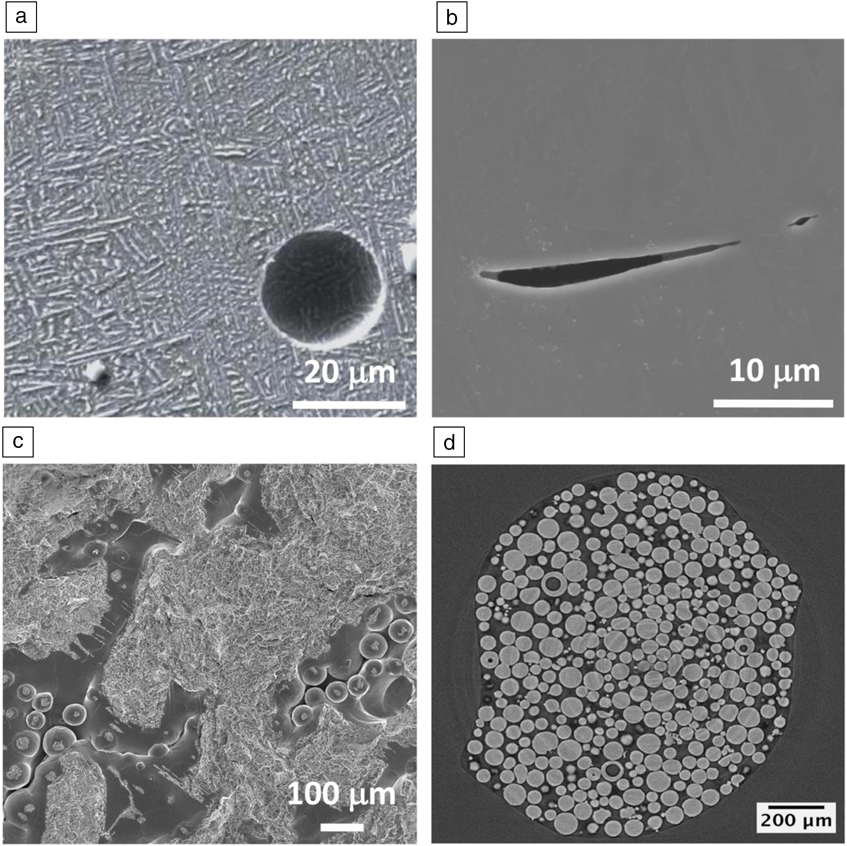
Figure 3. Examples of a gas pore (a) and a lack-of-fusion defect in selective electron-beam melting (SEBM)-fabricated (b) Ti-6Al-4V. Reference Lu, Tang, Ning, Liu, St. John and Qian23 (c) Unmelted and incompletely melted Ti-6Al-4V particles observed on an SEBM Ti-6Al-4V tensile fracture surface. Adapted with permission from Reference Reference Galarraga, Lados, Dehoff, Kirka and Nandwana27. © 2016 Elsevier. (d) Synchrotron x-ray microtomograph of internal porosity in Arcam gas-atomized (GA) Ti-6Al-4V particles. Eight out of the 427 GA Ti-6Al-4V particles or 1.87% of the particles examined show internal porosity. Adapted with permission from Reference Reference Cunningham, Narra, Ozturk, Beuth and Rollett28. © 2016 Springer.
In general, the overall porosity or defects in AM Ti-6Al-4V can be controlled to be less than 0.3 vol%, Reference Tammas-Williams, Zhao, Léonard, Derguti and Todd29–Reference Becker, van Rooyen and Dimitrov32 but current requirements demand ensuring this to be less than 0.1 vol% at present. A variety of factors can affect the formation of defects. In addition to the AM routes (e.g., scanning patterns) and parameters, the occurrence of gas-entrapped pores seems to be closely related to the feedstock quality. Svensson and Ackelid Reference Svensson, Ackelid and Gilbert30 reported that the use of gas-atomized (GA) Ti-6Al-4V powder containing 0.27 vol% porosity resulted in 0.19 vol% final porosity in SEBM Ti-6Al-4V samples, while the use of lower-porosity (0.17 vol%) GA powder led to lower final porosity (0.11 vol%). GA Ti-6Al-4V powder can contain noticeable internal porosity, as shown in Figure 3d, Reference Cunningham, Narra, Ozturk, Beuth and Rollett28 in which eight out of the 427 GA Ti-6Al-4V particles (1.87%) show gas-entrapped pores.
Ti-6Al-4V powder produced by the plasma-rotating electrode process (PREP) is known to contain much less porosity (0.017 vol%) Reference Ahsan, Pinkerton, Moat and Shackleton18 than GA Ti-6Al-4V powder. The use of PREP powder is therefore expected to favor lower final porosity. However, it is challenging to produce fine PREP Ti-6Al-4V powder (≤45 μm) due to both the low density of molten Ti-6Al-4V (4.12 g cm–3 at 1700°C) Reference Li, Johnson and Rhim33 and the maximum rotation speed that can be realized (≤18,000 rpm).
Other factors that may contribute to the final porosity include evaporation of the low-melting-point Al due to the high-temperature melt pool (2000–2500°C), which may lead to Al vapor-entrapped pores, and the reduced solubility of gas in the melt pool from 2500°C to 1650°C (liquidus). Modeling and simulations have shown that dynamic flow patterns in the melt pool (caused by a variety of factors) can also affect the porosity formation process. Reference Khairallah, Anderson, Rubenchik and King34
It is important to note that even though the porosity in AM Ti-6Al-4V can be controlled to be as low as 0.1 vol%, the number of damaging pores can still be significant. To illustrate this, consider a 10-mm diameter and 100-mm long AM Ti-6Al-4V rod containing 0.1 vol% porosity. Assume that all of the pores are spherical and each pore is 30 μm in diameter, which is large enough to be damaging for high-cycle fatigue (HCF) performance. Reference Saito and Furuta35 The presence of 0.1 vol% porosity means that, on average, there exist 5555 pores in each millimeter-thick slice of the rod for a spatially uniform pore distribution. Nonuniform pore distributions can be more damaging. The lack-of-fusion defects and large gas-entrapped pores are thus of real concern in fatigue critical components.
Tensile properties of AM Ti-6Al-4V
Ti-6Al-4V is most commonly used in the mill-annealed condition. However, Ti-6Al-4V can be hardened in sections up to 25.4 mm thickness via a solution treatment and aging (STA) process. 36 Table I lists the minimum tensile properties of mill-annealed and STA Ti-6Al-4V at room temperature as a point of reference for the discussion of the tensile properties of AM Ti-6Al-4V.
Table I. Minimum tensile properties of mill-annealed and solution-treated and aged (STA) Ti-6Al-4V at room temperature (YS, yield strength; UTS, ultimate tensile strength).
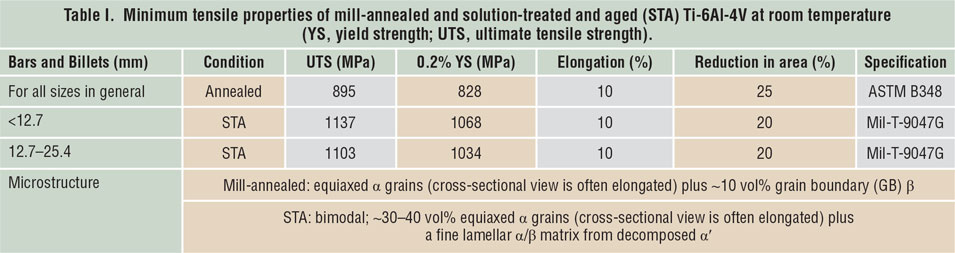
Laser AM Ti-6Al-4V and effects of heat treatment
The following discussion is based on two recent reviews of the mechanical properties of laser AM Ti-6Al-4V. Reference Beese and Carroll37,Reference Bian, Thompson and Shamsaei38 Figure 4 summarizes the tensile properties of AM Ti-6Al-4V available from literature, Reference Al-Bermani, Blackmore, Zhang and Todd39–43 with Figure 4a focusing on laser AM and Figure 4b on SEBM in both the as-built and heat-treated conditions. The heat treatments involved cover commonly used sub-transus (i.e., below ∼996°C in the α-β region) and super-transus (i.e., above ∼996°C in the β region) heat-treating processes for Ti-6Al-4V, as well as hot isostatic pressing (HIP), which simultaneously applies high gas pressure (usually with argon) to reduce porosity and heal microcracks. The β transus of Ti-6Al-4V varies in the range 996 ± 14°C, depending on the actual Al (5.5–7.5) and V (3.5–4.5) contents.
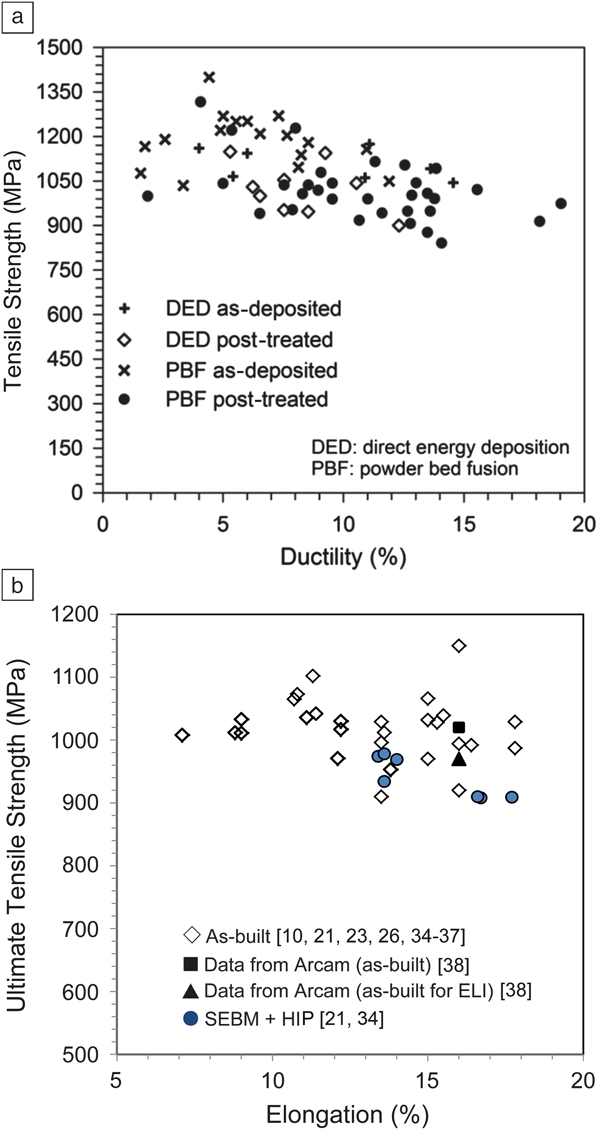
Figure 4. Tensile properties of additively manufactured Ti-6Al-4V with and without post-AM heat treatments: (a) Laser-based AM. Adapted with permission from Reference Reference Beese and Carroll37. © 2016 Springer. (b) Selective electron-beam melting (SEBM) results from various studies, including samples that have undergone hot isostatic pressing (HIP). These tensile properties may have been affected by variation in the oxygen content of the powders used; for example, Arcam Ti-6Al-4V powder contains 0.15 wt% oxygen, while Arcam Ti-6Al-4V extra-low interstitial (ELI) powder contains 0.10 wt%.
The following observations are notable comparing Figure 4a with Table I and other literature data on laser AM Ti-6Al-4V. Reference Beese and Carroll37–Reference Vilaro, Colin and Bartout47
-
• The tensile ductility of laser AM Ti-6Al-4V in the as-built condition, either by powder bed fusion or direct deposition, is often below the minimum requirement (10%) for mill-annealed Ti-6Al-4V, while the tensile strengths can easily meet the requirement.
-
• Applying sub-transus heat treatments above the effective martensite decomposition temperature (780°C) Reference Huang, Liu, Yang, Zhang, Shen and Feng44 to SLM Ti-6Al-4V can increase tensile ductility, but to achieve greater than 10% tensile elongation, it is generally necessary to anneal the SLM Ti-6Al-4V at temperatures ≥940°C, followed by either furnace cooling or air cooling. Reference Vrancken, Thijs, Kruth and Van Humbeeck45,Reference Thöne, Leuders, Riemer, Tröster and Richard46 Water quenching is not favored, as the resulting martensite (α′) structure is much coarser (∼1–2-μm-thick α′ laths) than the initial SLM α′ structure (300–500-nm-thick α′ laths). Reference Vilaro, Colin and Bartout47 Post-AM heat treatments below 780°C generally fail to show any noticeable influence on the tensile properties of AM Ti-6Al-4V. Reference Becker, van Rooyen and Dimitrov32,Reference Khairallah, Anderson, Rubenchik and King34,43
-
• Super-transus heat treatments can increase the tensile elongation to above 10% under air or furnace cooling conditions, with or without subsequent stabilization annealing. Reference Beese and Carroll37 In addition, it can transform the columnar prior-β grains into equiaxed β grains through a proposed shearing mechanism, which appears to occur only at high cooling rates. Reference Vilaro, Colin and Bartout47 However, the actual contribution of this solid-state columnar-to-equiaxed transition to mechanical properties remains unclear, because noticeable grain coarsening occurs at super-transus temperatures.
-
• AM Ti-6Al-4V exhibits anisotropy in both tensile strength and ductility and the trend of anisotropy can be opposing for strength and ductility. Reference Vilaro, Colin and Bartout47–Reference Zhao, Li, Zhang, Liu, Sercombe, Wang, Hao, Yang and Murr49 When inclined walls or struts are present in an AM Ti-6Al-4V structure, their properties may differ in the vertical and horizontal orientations. Post-AM sub-transus (e.g., 950°C/1 h) or super-transus (e.g., 1050°C/1 h) heat treatments can mitigate the degree of anisotropy, but it is difficult to eliminate it.
-
• Pronounced distortion has been observed for laser-powder-deposited large Ti-6Al-4V structures (1.1 m long) after unclamping them in the as-built state due to relief of residual stresses. Reference Qiu, Ravi, Dance, Ranson, Dilworth and Attallah11 Residual stresses were measured to be ~300 MPa in simple as-built SLM Ti-6Al-4V samples. Reference Simonelli, Tse and Tuck48 In order to avoid significant distortion, large or intricate laser AM Ti-6Al-4V structures need to be heat treated in a properly clamped form inherited from the as-fabricated state, or subjected to a proper stress-relief annealing treatment.
Electron-beam AM Ti-6Al-4V and effects of heat treatments
Compared with laser AM Ti-6Al-4V, SEBM Ti-6Al-4V can readily satisfy the minimum tensile property requirements for mill-annealed Ti-6Al-4V (see Figure 4b), although occasionally, the tensile elongation may fail to reach 10%. As a result, post-AM heat treatments of SEBM Ti-6Al-4V (excluding HIP) have not received much attention. Large SEBM Ti-6Al-4V structures can be used in the as-built state for non-fatigue critical applications, such as a recently developed honeycomb-structured SEBM Ti-6Al-4V oil-gas separation rotor. Reference Tang, Wang, Yang, Gu, Liu, Jia and Qian50 Additionally, owing to the relatively high SEBM powder bed temperatures (the substrate is often set at ∼730°C; substrate temperatures up to 1100°C were used for SEBM of TiAl), Reference Tang, Yang, Jia, He, Lu and Qian51 post-AM stress relief annealing is usually unnecessary for SEBM Ti-6Al-4V. Anisotropy in tensile strengths or ductility also occurs in SEBM Ti-6Al-4V, but to a lesser degree in general than in laser AM Ti-6Al-4V. Reference Galarraga, Lados, Dehoff, Kirka and Nandwana27,Reference Bian, Thompson and Shamsaei38
HIP pressing of AM Ti-6Al-4V
HIP of AM Ti-6Al-4V is typically carried out at 920°C for 2 h under 100 MPa in argon, 43 which can close or heal internal pores and lack-of-fusion defects to below the resolution limit of x-ray micro-computer tomography (∼5 μm Reference Tammas-Williams, Withers, Todd and Prangnell52 ). The use of higher-resolution synchrotron x-ray microtomography (1.5 μm Reference Cunningham, Narra, Ozturk, Beuth and Rollett28 ) is expected to further clarify the effectiveness of HIP in closing small pores. Columnar grain boundaries remain after HIP at 920°C. However, owing to the nearly pore-free and equilibrium HIP microstructures, the tensile ductility increases to above 10% for laser AM Ti-6Al-4V, while for SEBM-HIP Ti-6Al-4V, tensile elongation can reach more than 16%. Reference Lu, Tang, Ning, Liu, St. John and Qian23 A downside is that AM-HIP Ti-6Al-4V could end up with yield strength below the minimum requirement of 828 MPa (Table I) due to the coarse lamellar α/β and grain boundary α developed during HIP.
The classical Hall–Petch relationship approximately applies to AM Ti-6Al-4V in terms of yield strength varying linearly with the inverse square root of α-lath thickness ( Figure 5 ). The α-lath thickness corresponding to the minimum yield strength requirement of 828 MPa is about 8.0 μm (Figure 5). This prediction generally agrees with the coarse lamellar α/β structures of AM-HIP Ti-6Al-4V that show yield strengths below 828 MPa. Reference Lu, Tang, Ning, Liu, St. John and Qian23 Consequently, other HIP conditions may need to be explored. The microstructure–property connection shown in Figure 5 served as the roadmap for the recent development of STA-grade SLM Ti-6Al-4V (to be discussed subsequently).

Figure 5. Tensile yield strength versus inverse square root of α-lath thickness for lamellar α/β Ti-6Al-4V. The filled square (blue) corresponds to as-built selective laser melting Ti-6Al-4V with an ultrafine lamellar α/β microstructure (Figure 2b, α-lath thickness: ∼300 nm), which attained tensile strength >1200 MPa, yield strength >1100 MPa, and tensile elongation >10%. Adapted with permission from Reference Reference Xu, Brandt, Sun, Elambasseril, Liu, Latham, Xia and Qian17. © 2015 Elsevier.
Effects of as-built surface conditions
The tensile properties of AM Ti-6Al-4V previously discussed were obtained from either machined (average roughness R a ≈ 1 μm) or polished (R a ≈ 0.1–0.5 μm) samples. AM Ti-6Al-4V shows relatively rough surfaces, similar to sand castings (R a ≈ 12.5–25 μm). 53 For example, SLM Ti-6Al-4V surfaces typically have R a ≈ 10–25 μm, while SEBM Ti-6Al-4V surfaces are usually rougher with R a up to 40 μm. Reference Sun, Gulizia, Oh, Fraser, Leary, Yang and Qian54 Consequently, as-built AM Ti-6Al-4V specimens show noticeably lower tensile strengths and ductility, irrespective of the AM process, Reference de Formanoir, Michotte, Rigo, Germain and Godet19,Reference Sun, Gulizia, Oh, Fraser, Leary, Yang and Qian54–Reference Greitemeier, Palm, Syassen and Melz58 as microcracks were found to directly initiate from surface defects. Reference Sun, Gulizia, Oh, Fraser, Leary, Yang and Qian54,Reference Alcisto, Enriquez, Garcia, Hinkson, Steelman, Silverman, Valdovino, Gigerenzer, Foyos, Ogren and Dorey57 Acid etching, surface polishing, and machining have all proved to be effective in improving the surface roughness and therefore the mechanical properties. Abrasive fluid machining (AFM) can be another option, although it is more costly. For SEBM Ti-6Al-4V, it appears necessary to remove a ∼650-μm-thick surface in order to eliminate the detrimental surface effect. Reference Palanivel, Dutt, Faierson and Mishra55 This can be problematic for AM of Ti-6Al-4V components with thin internal sections or struts in lattice structures. In addition, the detrimental influence of the as-built surface conditions on tensile mechanical properties remains after HIP or annealing for both SEBM Ti-6Al-4V and laser powder fusion Ti-6Al-4V. Reference Greitemeier, Palm, Syassen and Melz58 Post-AM surface treatments are thus important to ensure good mechanical properties, regardless of the subsequent heat-treatment process.
As AM technology continues to develop, improved surface conditions, which depend on both the feedstock materials and the AM process, will improve the performance consistency as well as the mechanical properties of the as-built AM Ti-6Al-4V parts. This will be particularly useful for the AM of intricate Ti-6Al-4V components designed for load-bearing applications, due to the difficulty of modifying their internal surfaces, even by acid etching or AFM.
Fatigue properties of AM Ti-6Al-4V
As with other metallic alloys, the fatigue performance of AM Ti-6Al-4V depends on surface conditions, microstructure, and internal defects. No HCF requirements are specified in the ASTM standards for Ti-6Al-4V. Table II Reference Tammas-Williams, Zhao, Léonard, Derguti and Todd29,Reference Grylls59–Reference Baufeld, Brandl and Van der Biest64 lists typical fatigue strengths of non-AM Ti-6Al-4V and the achievable fatigue strengths of AM Ti-6Al-4V in the as-built condition (machined surfaces) by SLM, SEBM, and LMD. Most of the fatigue data reported on AM Ti-6Al-4V in both the as-built and heat-treated conditions have been assessed in two recent reviews. Reference Bian, Thompson and Shamsaei38,Reference Li, Warner, Fatemi and Phan65 The following observations are prominent from these assessments Reference Bian, Thompson and Shamsaei38,Reference Li, Warner, Fatemi and Phan65 in conjunction with other fatigue data reported on AM Ti-6Al-4V. Reference Tammas-Williams, Zhao, Léonard, Derguti and Todd29,Reference Greitemeier, Palm, Syassen and Melz66,Reference Åkerfeldt, Pederson and Antti67
-
• Post-AM surface treatments are essential to achieve superior fatigue performance. For example, the fatigue strength of SLM Ti-6Al-4V specimens (Ra ≈ 13 μm) was 210 MPa, compared to 500 MPa for polished SLM Ti-6Al-4V specimens (Ra ≈ 0.5 μm). Reference Wycisk, Solbach, Siddique, Herzog and Walther63 In addition, shot peening, which entails impacting the surface with round steel particles, has also proved to be effective in improving the fatigue strengths of AM Ti-6Al-4V due to the introduction of a compressive residual stress layer. Reference Wycisk, Emmelmann, Siddique and Walther68
-
• The fatigue strength data of AM Ti-6Al-4V scatter broadly. On the one hand, research has shown that AM Ti-6Al-4V, irrespective of the AM process, can achieve fatigue strengths that are comparable to, or even better than, those of the mill-annealed Ti-6Al-4V (Table II). On the other hand, AM Ti-6Al-4V can be inferior to as-cast Ti-6Al-4V.
-
• Anisotropic fatigue performance is observed in AM Ti-6Al-4V (Table II) due to the columnar prior-β grain structures, nonuniform distribution of pores and lack-of-fusion defects, and other microstructural features. Reference Tammas-Williams, Zhao, Léonard, Derguti and Todd29,Reference Bian, Thompson and Shamsaei38
-
• HIP can improve the fatigue strength and HCF performance consistency of AM Ti-6Al-4V because of much reduced internal porosity and the elimination of other defects. In general, Reference Bian, Thompson and Shamsaei38,Reference Li, Warner, Fatemi and Phan65 AM-HIP Ti-6Al-4V samples with machined surfaces can show HCF performance (≥550 MPa at 107 cycles Reference Greitemeier, Palm, Syassen and Melz58 ) fully comparable to, or better than, mill-annealed Ti-6Al-4V. In addition, AM-HIP Ti-6Al-4V exhibits a much smaller degree of anisotropy in fatigue strength than the conventionally heat-treated AM Ti6Al-4V, Reference Bian, Thompson and Shamsaei38,Reference Wu and Lai69 indicating that internal porosity and defects are largely responsible for fatigue anisotropy.
-
• Appropriate post-AM heat treatments can improve the fatigue strength of AM Ti-6Al-4V. However, since pressureless heat treatments are ineffective to shrink or close pores, HIP is necessary to ensure consistent HCF performance. A potential risk, although not demonstrated yet, is that lack-of-fusion defects and gas pores healed or shrunk by HIP may reopen after a certain number of loading and unloading cycles. A related study has shown that HIP-shrunk pores in SEBM Ti-6Al-4V can grow and reappear during subsequent β annealing due to the high internal argon gas pressure. Reference Tammas-Williams, Withers, Todd and Prangnell70 The argon gas arises from that entrapped in the GA powder as SEBM operates in high vacuum. Again, this shows the necessity of using pore-free powder feedstock for AM of critical parts. SLM Ti-6Al-4V may exhibit a similar or even worse scenario as argon is used as the protective gas during SLM. Wrought Ti-6Al-4V products do not normally have this latent risk.
Table II. Fatigue strengths of conventionally manufactured and additively manufactured Ti-6Al-4V tested at R = 0.1 (where R is the ratio of minimum to maximum peak stress).
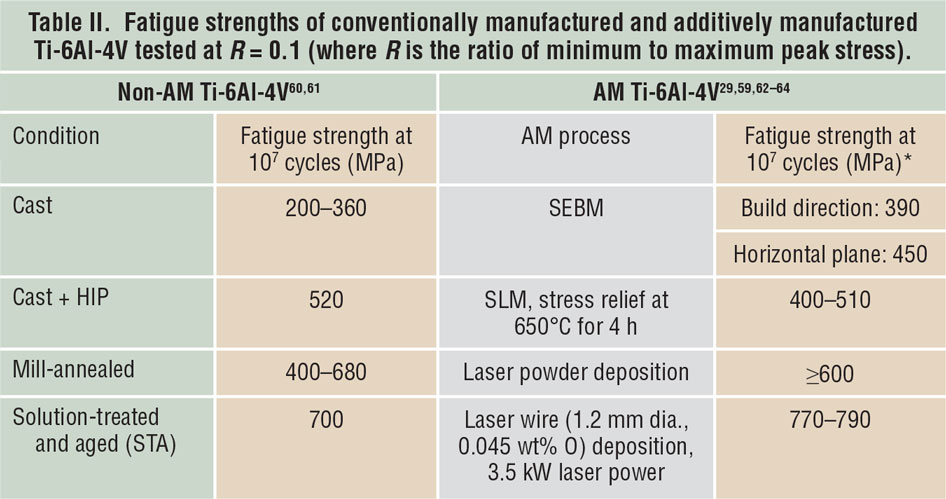
* Best fatigue strength data reported in literature for each AM process.
AM of STA-grade Ti-6Al-4V
Ti-6Al-4V in the STA condition offers the highest strength of the alloy Ti-6Al-4V while still possessing reasonable ductility (Table I). Therefore, it has been used for a variety of applications. The bimodal microstructure of the STA Ti-6Al-4V (globular α + fine lamellar α/β) is, however, not easily achievable by current AM processes. Reference Simonelli, Tse and Tuck71
A recent development has shown that STA-grade tensile mechanical properties are achievable by AM without having to produce a bimodal microstructure. Reference Xu, Brandt, Sun, Elambasseril, Liu, Latham, Xia and Qian17 In this new development, the as-built SLM Ti-6Al-4V attained tensile strength >1200 MPa, yield strength >1100 MPa, and tensile elongation >10% (the filled square shown in Figure 5). Reference Xu, Brandt, Sun, Elambasseril, Liu, Latham, Xia and Qian17 This was realized by taking advantage of the pronounced formation of martensite in SLM Ti-6Al-4V and allowing the martensite to in situ decompose into ultrafine lamellar α/β (Figure 2b, α-lath thickness: ∼300 nm) during AM. Reference Xu, Brandt, Sun, Elambasseril, Liu, Latham, Xia and Qian17 As shown in Figure 5, Reference Xu, Brandt, Sun, Elambasseril, Liu, Latham, Xia and Qian17,Reference Al-Bermani, Blackmore, Zhang and Todd39,Reference Hrabe and Quinn42,Reference Vrancken, Thijs, Kruth and Van Humbeeck45,Reference Vilaro, Colin and Bartout47,Reference Hrabe and Quinn72–Reference Facchini, Magalini, Robotti, Molinari, Hoges and Wissenbach76 ultrafine α laths correspond to high yield strengths. The resulting ultrafine lamellar α-β structure also exhibited good fatigue strength (400 MPa) in the as-built state, Reference Xu, Sun, Elambasseril, Liu, Brandt and Qian77 although it is still lower than that of the STA Ti-6Al-4V (700 MPa, Table II).
Research is under way to apply this martensite-decomposition-based approach to the AM of Ti-6Al-4V parts with different section thicknesses. Factors that affect the formation and decomposition of martensite in both SLM and SEBM Ti-6Al-4V have received increasing attention for improved microstructural control during AM. Reference Yang, Yu, Yin, Gao, Wang and Zeng78,Reference Tan, Kok, Toh, Tan, Descoins, Mangelinck, Tor, Leong and Chua79 In addition, unlike martensitic transformation by which martensite laths always form in the parent β grains without changing the parent grain structure, massive transformation in AM Ti-6Al-4V leads to entirely new massive αm grains (see Figure 2d). Reference Lu, Qian, Tang, Yan, Wang and St. John24 This offers a new strategy to transform the columnar β grains in AM Ti-6Al-4V into equiaxed αm grains. Research has shown that, similar to martensite, αm can also decompose into ultrafine α/β structures in AM Ti-6Al-4V. Reference Lu, Qian, Tang, Yan, Wang and St. John24 The β → αm transformation thus offers a different pathway to microstructural and alloy designs for AM. Further microstructural innovations have been demonstrated on other AM titanium alloys by taking advantage of the self-aging process during AM (e.g., the realization of 50–250 nm Y2O3 precipitates in a SEBM titanium alloy Ti-6Al-2.7Sn-4Zr-0.4Mo-0.45Si-0.1Y). Reference Lu, Tang, Qian, Zeng and St. John80
Concluding remarks
Extensive research performed during the last decade on AM of Ti-6Al-4V alloy has been instrumental in demonstrating the attributes and potential of metal AM technology for industrial applications. AM Ti-6Al-4V in the as-built state (with machined or polished surfaces) can be made to be fully comparable to, or even better than, wrought-grade (mill-annealed) Ti-6Al-4V in terms of both tensile and fatigue performance. However, the mechanical properties can show a large degree of scatter, especially the fatigue strength data, and are often anisotropic. AM build orientation affects both the degree of scatter and the degree of anisotropy.
Pressureless normal post-AM heat treatments in general are not needed for SEBM Ti-6Al-4V, but they are necessary for laser AM Ti-6Al-4V for improved ductility and reduced anisotropy in mechanical properties. Porosity and lack-of-fusion defects remain a major concern, which not only downgrades mechanical performance, but also entails inconsistent and anisotropic responses. Current AM practice with GA Ti-6Al-4V powder can achieve about 99.9% relative density. However, even with 0.1 vol% porosity, the number of damaging pores can still be significant, particularly for fatigue-critical applications. It is desirable to use pore-free powder feedstock providing that neither the availability nor the affordability is an issue.
HIP is a necessary backup to heal or shrink both the internal porosity and lack-of-fusion defects in AM Ti-6Al-4V parts, but there is a potential risk that HIP-shrunk pores may reappear under certain circumstances. The as-built surface conditions exert a noticeable influence on both the tensile and fatigue performance, and the detrimental influence remains after HIP or annealing treatments. Appropriate post-AM surface treatments are necessary for AM Ti-6Al-4V components. Innovative microstructural designs, including design of the pathways to their realization within the specification of Ti-6Al-4V, will continue to hold the key to the development of STA-grade AM Ti-6Al-4V in the as-built state. Further improvements in current AM processes including the feedstock quality and forms that can lead to the fabrication of pore-free AM Ti-6Al-4V can be another major milestone development for the future of metal AM.
Acknowledgments
The authors thank the Australian Research Council (ARC) and National Natural Science Foundation of China (NSFC) for their financial support through ARC DP150104719, ARC LP140100607, and NSFC No. 51528401. They also thank S.L. Lu for useful discussions.