1. Introduction
The Central Asian Orogenic Belt (CAOB) (also known as the Altaid Tectonic Collage) is the world’s largest Palaeozoic accretionary orogen (Fig. 1). The CAOB is related to the closure of the Palaeo-Asian Ocean and represents a significant region of Phanerozoic crustal growth (Coleman, Reference Coleman1989; Sengör et al. Reference Sengor, Natalin and Burtman1993; Jahn et al. Reference Jahn, Wu and Chen2000, Reference Jahn, Capdevila, Liu, Vernon and Badarch2004; Windley et al. Reference Windley, Alexeiev, Xiao, Kroner and Badarch2007; Wilde et al. Reference Wilde, Wu and Zhao2010; Xu et al. Reference Xu, Ji, Zhao, Gong, Zhou, He, Zhong, Wang and Griffiths2013). It is widely accepted that the CAOB is related to the closure of the Palaeo-Asian Ocean from the Early Neoproterozoic to the Late Palaeozoic (Jahn et al. Reference Jahn, Wu and Chen2000; Chen & Jahn, Reference Chen and Jahn2004; Han et al. Reference Han, Ji, Song, Chen and Zhang2006; Windley et al. Reference Windley, Alexeiev, Xiao, Kroner and Badarch2007; Xiao & Santosh, Reference Xiao and Santosh2014). This closure involved the accretion of oceanic islands, seamounts, oceanic plateaus, island arcs, accretionary complexes, ophiolites and microcontinents to the southern margin of the Siberian Craton (Feng et al. Reference Feng, Coleman, Tilton and Xiao1989; Windley et al. Reference Windley, Alexeiev, Xiao, Kroner and Badarch2007; Xiao et al. Reference Xiao, Han, Yuan, Sun and Lin2008, Reference Xiao, Huang, Han, Sun and Li2010; Zhang et al. Reference Zhang, Wang, Shen, Polat and Zhu2021a, Reference Zhang, Liu, Xiao, Xu, Shi, Gong, Hu, Liu, Song, Xiao, Zhang, Li and Li2021b).
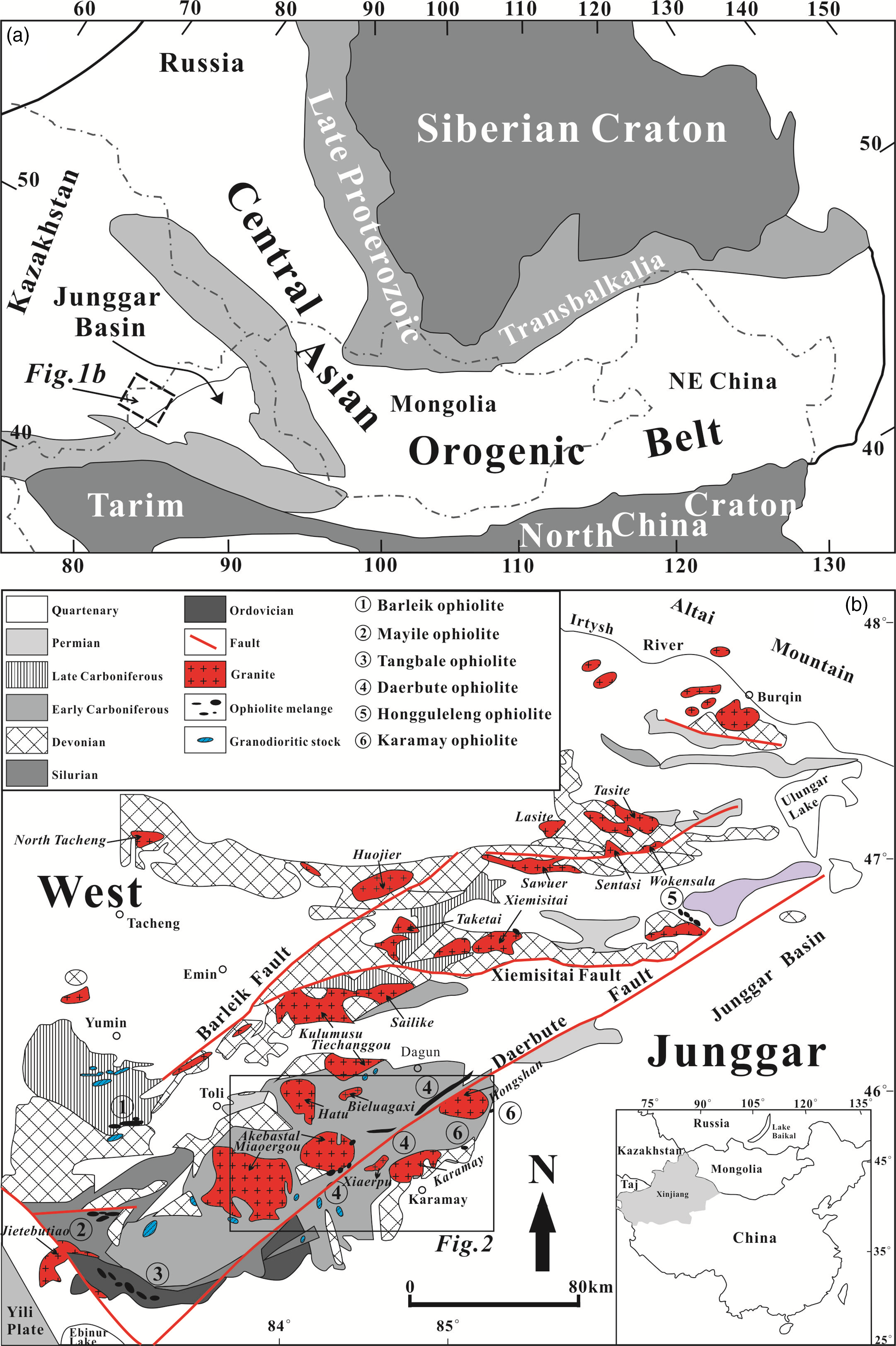
Figure 1. (a) Simplified tectonic map of the Central Asian Orogenic Belt (Sengör et al. Reference Sengor, Natalin and Burtman1993). (b) Simplified geological map of the West Junggar (modified after Feng et al. Reference Feng, Coleman, Tilton and Xiao1989), inset shows the position of Xinjiang in NW China.
The West Junggar region, in the southwestern part of the CAOB, plays a crucial role in understanding the Palaeozoic tectonic evolution and crustal growth within the orogenic belt (Windley et al. Reference Windley, Alexeiev, Xiao, Kroner and Badarch2007; Xiao et al. Reference Xiao, Han, Yuan, Sun and Lin2008; Choulet et al. Reference Choulet, Faure, Cluzel, Chen, Lin and Wang2012; Xu et al. Reference Xu, Ji, Zhao, Gong, Zhou, He, Zhong, Wang and Griffiths2013). Both lateral accretion in active continental margins and vertical underplating at the crust-mantle interface contributed to continental crustal growth in CAOB (Jahn et al. Reference Jahn, Wu and Chen2000; Windley et al. Reference Windley, Alexeiev, Xiao, Kroner and Badarch2007). Voluminous Late Palaeozoic granitoids with high and positive εNd(t) values were intruded throughout West Junggar (Jahn et al. Reference Jahn, Wu and Chen2000; Chen & Jahn, Reference Chen and Jahn2004; Han et al. Reference Han, Ji, Song, Chen and Zhang2006) and include a range of magmatic rocks including high-Mg andesites (e.g., sanukitoids/sanukitic rocks, adakites/adakitic rocks), A- and I-type granites and Nb-enriched diorites (Chen et al. Reference Chen, Han, Ji, Zhang, Xu, He and Wang2010; Yin et al. Reference Yin, Yuan, Sun, Long, Zhao, Wong, Geng and Cai2010, Reference Yin, Chen, Xiao, Yuan, Sun, Tang, Yu, Long, Cai, Geng, Zhang and Liu2015a; Tang et al. Reference Tang, Wang, Wyman, Li, Xu and Zhao2012a, Reference Tang, Wang, Wyman, Li, Zhao and Yang2012b; Xu et al. Reference Xu, Ji, Zhao, Gong, Zhou, He, Zhong, Wang and Griffiths2013; Zhang et al. Reference Zhang, Wang, Polat, Zhu, Shen, Chen, Chen, Guo, Wu and Liu2018, Reference Zhang, Wang, Shen, Polat and Zhu2021a; Zheng et al. Reference Zheng, Han, Wang, Liu and Feng2020). However, the tectono-magmatic setting of these Late Carboniferous to Permian magmatic rocks in West Junggar remains controversial with a range of tectonic settings and processes being proposed. These include: post-collisional (Chen & Jahn, Reference Chen and Jahn2004; Han et al. Reference Han, Ji, Song, Chen and Zhang2006; Chen et al. Reference Chen, Han, Ji, Zhang, Xu, He and Wang2010; An et al. Reference An, Zhu, Wei, Zhang and Zhao2022b), mantle plume (Gao et al. Reference Gao, Xiao, Pirajno, Wang, He, Yang and Yan2014), remnant oceanic basin (Xu et al. Reference Xu, Ji, Zhao, Gong, Zhou, He, Zhong, Wang and Griffiths2013; Zhang et al. Reference Zhang, Wang, Polat, Zhu, Shen, Chen, Chen, Guo, Wu and Liu2018), subduction-accretion (Xiao et al. Reference Xiao, Han, Yuan, Sun and Lin2008, Reference Xiao, Huang, Han, Sun and Li2010) and ridge subduction (Geng et al. Reference Geng, Sun, Yuan, Xiao, Xian, Zhao, Zhang, Wong and Wu2009; Tang et al. Reference Tang, Wang, Wyman, Li, Zhao, Jia and Jiang2010, Reference Tang, Wang, Wyman, Li, Xu and Zhao2012a, Reference Tang, Wang, Wyman, Li, Zhao and Yang2012b; Yin et al. Reference Yin, Long, Yuan, Sun, Zhao and Geng2013, Reference Yin, Chen, Yuan, Yu, Xiao, Li and Sun2015b, Reference Yin, Chen, Xiao, Yuan, Sun, Tang, Yu, Long, Cai, Geng, Zhang and Liu2015a; Windley & Xiao, Reference Windley and Xiao2018) processes.
Intermediate-mafic dykes are widespread in the southern West Junggar (Fig. 1), especially in the Karamay, Xiaerpu, Bieluagaxi and Liushugou areas (Fig. 2), which provide an excellent opportunity to understand the geological evolution of the West Junggar. The dykes in West Junggar are referred to as high-magnesium andesites (HMAs) including sanukitoids and adakitic-like rocks (Yin et al. Reference Yin, Yuan, Sun, Long, Zhao, Wong, Geng and Cai2010, Reference Yin, Long, Yuan, Sun, Zhao and Geng2013; Duan et al. Reference Duan, Li, Zhi, Yang and Gao2019), which are distinct from typical island arc andesites due to their elevated SiO2, MgO and Mg# (Mg# = Mg2+/(Mg2++Fe2+)), Cr and Ni contents (Tatsumi, Reference Tatsumi2001). Their geochemical and isotopic features reveal an affinity of both mantle and crust and an uncommon tectonic setting in association with subduction zones or post-collisional environment (Qian & Hermann, Reference Qian and Hermann2010). Three major alternative models have been proposed to account for the geochemical characteristics of these West Junggar dykes: (a) partial melting of peridotites metasomatized by slab-derived melts/fluids (Yin et al. Reference Yin, Yuan, Sun, Long, Zhao, Wong, Geng and Cai2010, Reference Yin, Long, Yuan, Sun, Zhao and Geng2013, Reference Yin, Chen, Xiao, Yuan, Sun, Tang, Yu, Long, Cai, Geng, Zhang and Liu2015a, Reference Yin, Chen, Yuan, Yu, Xiao, Li and Sun2015b; Tang et al. Reference Tang, Wang, Wyman, Li, Xu and Zhao2012a, Reference Tang, Wang, Wyman, Li, Zhao and Yang2012b); or (b) sediment-derived melts at a ridge subduction environment (Duan et al. Reference Duan, Li, Zhi, Yang and Gao2019) or (c) partial melting of remnant oceanic crust formed in early Palaeozoic during post-collisional processes (Xu et al. Reference Xu, Ji, Zhao, Gong, Zhou, He, Zhong, Wang and Griffiths2013). Thus, detailed petrological, geochemical and geochronological studies of the dykes in this area could help to resolve which model is most appropriate. Intermediate-mafic dykes usually occur in tensional tectonic regimes, and record information on the timing and processes of continental break-up (Strvastava, 2011). They also can be used to reconstruct the paleostress field and provide information on the genesis and tectonic setting (Ma et al. Reference Ma, Xiao, Windley, Zhao, Han, Zhang, Luo and Li2012).

Figure 2. (a) Geological map of the study area (modified after Ma et al. Reference Ma, Xiao, Windley, Zhao, Han, Zhang, Luo and Li2012). Positions of Fig. 2b–d are marked. (b) Simplified geological map of the Karamay and Xiaerpu dykes (modified after Duan et al. Reference Duan, Li, Zhi, Yang and Gao2019). (c) Simplified geological map of the Liushugou dykes (modified after Yin et al. Reference Yin, Yuan, Sun, Long, Zhao, Wong, Geng and Cai2010). (d) Simplified geological map of the Bieluagaxi dykes (modified after Yin et al. Reference Yin, Chen, Xiao, Yuan, Sun, Tang, Yu, Long, Cai, Geng, Zhang and Liu2015a).
In this paper, we present zircon U-Pb ages, elemental and Sr-Nd isotopic data for the Carboniferous dykes in West Junggar (the Karamay, Xiaerpu, Bieluagaxi and Liushugou dykes) to explore their petrogenesis and tectonic implications during the Late Palaeozoic. Based on our new data, we propose that Carboniferous dykes in West Junggar are similar to HMAs and were likely formed by partial melting of peridotites metasomatised by remnant oceanic crust-derived melts or sediment-derived melts in a post-collisional setting.
2. Geological background
The CAOB (Fig. 1a) is located between the Siberian Craton to the north and the Tarim and North China Cratons to the south. It extends from the Urals in the west, through Uzbekistan, Tajikistan, Kazakhstan, NW China, Mongolia and NE China to the Okhotsk Sea in the Eastern Russia (Sengör et al. Reference Sengor, Natalin and Burtman1993; Jahn et al. Reference Jahn, Wu and Chen2000; Windley, et al. Reference Windley, Alexeiev, Xiao, Kroner and Badarch2007). The CAOB is generally thought to be related to the closure of the Palaeo-Asian Ocean because it is a composite terrane made up of diverse fragments including ancient microcontinents, arc terranes, oceanic volcanic islands, volcanic plateaus, oceanic crust and passive continental margin sequences (Coleman, Reference Coleman1989; Buckman & Aitchison, Reference Buckman and Aitchison2004; Windley et al. Reference Windley, Alexeiev, Xiao, Kroner and Badarch2007; Choulet et al. Reference Choulet, Faure, Cluzel, Chen, Lin and Wang2012).
West Junggar is located in the middle of the Kazakhstan orocline (Tang et al. Reference Tang, Wang, Wyman, Li, Zhao, Jia and Jiang2010; Ma et al. Reference Ma, Xiao, Windley, Zhao, Han, Zhang, Luo and Li2012) and is surrounded by the Altai orogen to the north, the Tianshan orogen to the south, the Kazakhstan plate to the west, and the Junggar basin to the east (Fig. 1a). Numerous Cambrian to Carboniferous ophiolitic mélanges have been reported from West Junggar (Feng et al. Reference Feng, Coleman, Tilton and Xiao1989; He et al. Reference He, Liu, Zhang and Xu2007; Yang et al. Reference Yang, Li, Gu, Yang, Tong and Zhang2012a, Reference Yang, Li, Santosh, Gu, Yang, Zhang, Wang, Zhong and Tong2012b; Zhu et al. Reference Zhu, Chen and Qiu2015). Ordovician-Quaternary sedimentary strata are widely distributed in this region, with Devonian-Carboniferous volcano-sedimentary rocks being particularly abundant (BGMRX, 1993). West Junggar can be divided into northern and southern parts by the Xiemisitai Fault (Xu et al. Reference Xu, Ji, Zhao, Gong, Zhou, He, Zhong, Wang and Griffiths2013; Yin et al. Reference Yin, Chen, Xiao, Yuan, Sun, Tang, Yu, Long, Cai, Geng, Zhang and Liu2015a), and some have further divided the southern part of West Junggar into central and southern domains, which recorded different subduction-accretion history (Xu et al. Reference Xu, Ji, Zhao, Gong, Zhou, He, Zhong, Wang and Griffiths2013; Chen et al. Reference Chen, Han, Zhang, Xu, Liu, Qu, Li, Yang and Yang2015). Relatively continuous Carboniferous strata covered by the Permian molasse and continental volcanic rocks with an angular unconformity are dominant in central and southern West Junggar (Zong et al. Reference Zong, Wang, Jiang and Gong2016).
Abundant widespread Palaeozoic magmatism and evidence for a prolonged orogenic event are notable characteristics of the West Junggar terrane. Three magmatic pulses have been recognised (Han et al. Reference Han, Ji, Song, Chen and Zhang2006; Chen et al. Reference Chen, Han, Ji, Zhang, Xu, He and Wang2010; Gao et al. Reference Gao, Xiao, Pirajno, Wang, He, Yang and Yan2014). The oldest Late Devonian – Early Silurian pulse comprises calc-alkaline plutons and volcanic rocks (Chen et al. Reference Chen, Han, Ji, Zhang, Xu, He and Wang2010, Reference Chen, Han, Zhang, Xu, Liu, Qu, Li, Yang and Yang2015; Choulet et al. Reference Choulet, Faure, Cluzel, Chen, Lin and Wang2012). The middle pulse is composed of Early Carboniferous granitoids, which are characterised by I-type plutons and adakitic rocks and Nb-enriched basalts. The youngest pulse, mostly formed between 310 Ma and 290 Ma, is dominated by A-type granites and sanukitoids, including monzogranite, alkali granite and charnockite (Han et al. Reference Han, Ji, Song, Chen and Zhang2006; Geng et al. Reference Geng, Sun, Yuan, Xiao, Xian, Zhao, Zhang, Wong and Wu2009; Chen et al. Reference Chen, Han, Ji, Zhang, Xu, He and Wang2010; Tang et al. Reference Tang, Wang, Wyman, Li, Zhao and Yang2012b).
Extensive Late Carboniferous – Early Permian mafic to intermediate dyke swarms are also found in central West Junggar (e.g., Baogutu, Hatu, Akbastal, Xiaerpu, Karamay, Hongshan, Tiechanggou areas) (Yin et al. Reference Yin, Yuan, Sun, Long, Zhao, Wong, Geng and Cai2010, Reference Yin, Long, Yuan, Sun, Zhao and Geng2013 Reference Yin, Chen, Xiao, Yuan, Sun, Tang, Yu, Long, Cai, Geng, Zhang and Liu2015a, Reference Yin, Chen, Yuan, Yu, Xiao, Li and Sun2015b; Ma et al. Reference Ma, Xiao, Windley, Zhao, Han, Zhang, Luo and Li2012; Tang et al. Reference Tang, Wang, Wyman, Li, Xu and Zhao2012a; He et al. Reference He, Xiao, Wang, Gao, Yang and Yan2015; Li et al. Reference Li, He and Fan2015; Zhan et al, Reference Zhan, Hou, Hari and Shu2015; Duan et al. Reference Duan, Li, Zhi, Yang and Gao2019) (Fig. 2a).
3. Petrological characteristics
The Karamay mafic-intermediate dykes intrude the Late Carboniferous Karamay granitoid and Carboniferous volcano-sedimentary strata (Fig. 2a). Dyke thicknesses range from 0.2 m to 20 m, and are mostly between 1.5 m and 3 m. Although some dykes are a few kilometres in length, most are less than 1 km (Fig. 3a) and mainly trend WNW-ESE (280°-310°) with several dykes trending NE-SW (30°-60°) (Fig. 2b). The NE-SW trending dykes are mainly diorites with minor diabase, whereas the WNW-ESE trending dykes are represented by dioritic porphyry. The NE-SW trending dykes cut across the WNW-ESE trending dykes. No chilled margins against the granitic rocks were observed, but in some places, baked margins can be observed (Fig. 3b). The dykes are generally undeformed and are slightly weathered and chloritized. Compositionally, the diabase exhibits a fine-grained ophitic texture (Fig. 4a) and consists of plagioclase (55–60% Footnote 1 ), clinopyroxene (25–30%), with minor biotite (0–5%), hornblende (0–5%) and magnetite (<5%). The diorite has a massive and fine to medium-grained granular texture and consists of plagioclase (50–55%), hornblende (15–20%), biotite (5–15%) with minor quartz (0–10%), pyroxene (0–5%) and accessory minerals including zircon, magnetite and apatite (Fig. 4b). The dioritic porphyry contains phenocrysts of plagioclase and/or hornblende (15–35%). The matrix (65–35%) is microcrystalline and is composed of plagioclase, hornblende and biotite with minor quartz, pyroxene and Ti-Fe oxides with an aphanitic texture.

Figure 3. Outcrop photos of dykes and their host plutons. (a) Dykes intruded into the Karamay pluton. (b) Baked and chilled margins on the contact boundary between the dyke and the host rocks. (c) Dyke swarms intruded into Xiaerpu pluton. (d) Granitic as enclaves surrounded by the dyke. (e) Dykes intruded Lower Carboniferous strata (abbreviated and marked as C strata in the photo) in Liushugou area. (f) Intrusive contact between a NE-SW dyke and the Lower Carboniferous strata. (g) Bieluagaxi diorite intruded by the dyke with plagioclase xenocrysts on the edge of the dyke. (h) The dykes and the diorite interpenetrated with each other, and dioritic enclave and disharmonic fold. (i) The straight magmatic bands as orientation of aligned hornblende showing magma flow in the host Bieluagaxi granitic rocks. (j) The straight and curved magmatic bands with injection folds and granitic enclaves in the host Bieluagaxi granitic rocks.
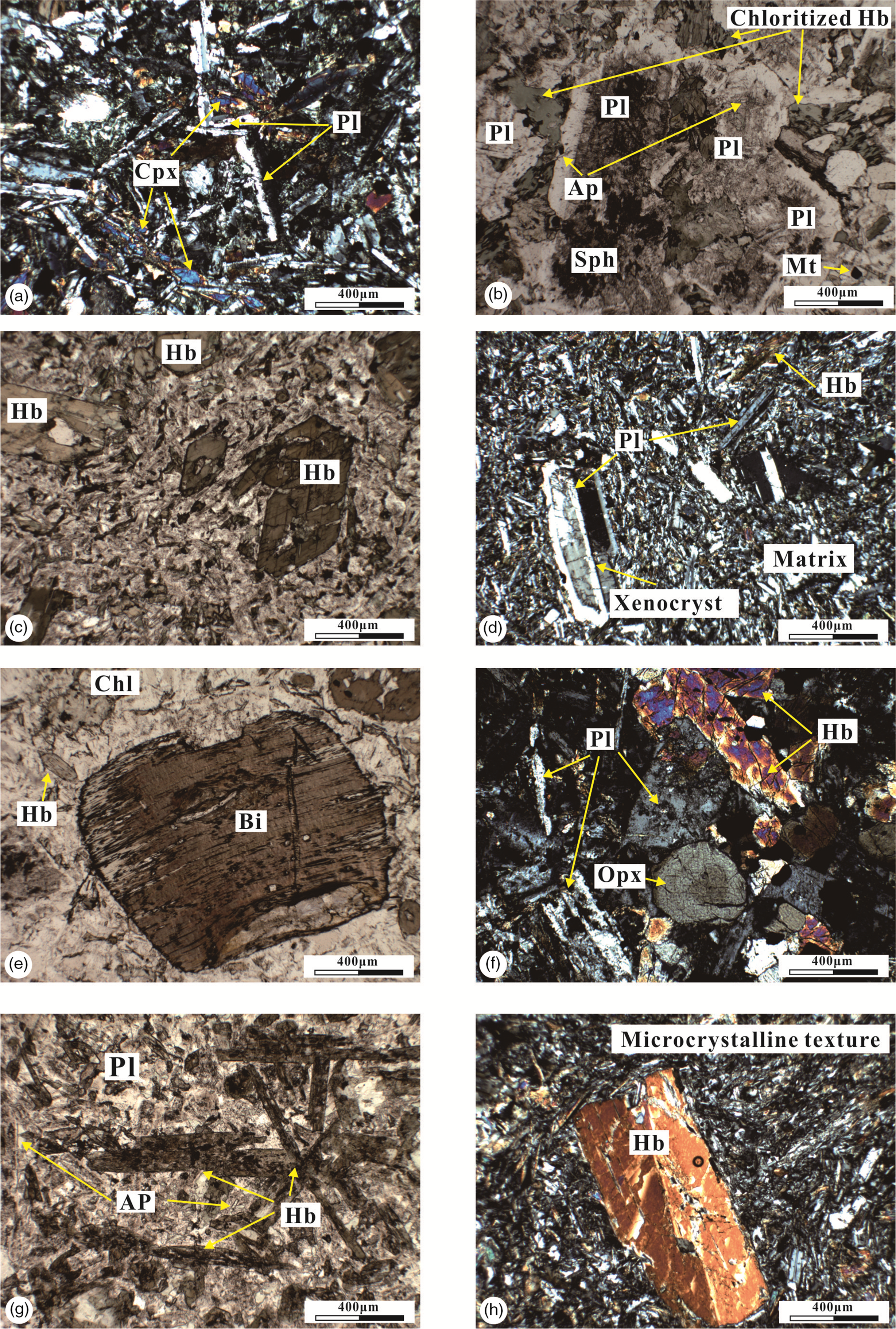
Figure 4. Microscopic photos of the dykes in the West Junggar. (a) Typical diabasic texture that xenomorphic clinopyroxenes filling in the spaces between the semi-euhedral acerose plagioclases, in Karamay dolerite dyke under the cross-polarised light. (b) Plagioclase, chloritized Hb with Ap, Sph and Mt in the Karamay dioritic dyke under the single-polarised light. (c) Porphyritic texture that phenocryst mainly consists of idiomorphic Hb crystals of the Xiaerpu dioritic porphyrite in the single-polarised light. (d) Mineral assemblages of the Xiaerpu dioritic porphyritic dyke in the cross-polarized light. Phenocryst consists of Pl and Hb, and the matrix shows microcrystalline texture with numerous acicular plagioclases and mafic minerals. (e) Hypidiomorphic sheet biotite as the phenocryst in dioritic porphyrite in Liushugou dioritic porphyrite in the single-polarised light. (f) Fine-grained granular texture with mineral assemblages of Pl, Hb and Opx in the Liushugou dioritic dyke under the cross-polarised light. (g) Long columnar Hb and plate-shaped Pl in the Bieluagaxi dioritic dyke under the single-polarised light. The euhedral amphiboles and acicular apatites embedded in the plagioclase indicate that the magma of dioritic dyke was initially quenched into the unconsolidated host granitic magma and then slowly cooled with it. (h) Porphyritic texture with twining Hb as phenocryst in the Bieluagaxi dioritic porphyritic dyke in the cross-polarised light. Pl = plagioclase, Opx = orthopyroxene, Cpx = Clinopyroxene, Hb = hornblende, Bi = biotite, Ap = apatite, Chl = chlorite, Sph = sphene, Mt = magnetite.
Xiaerpu intermediate to mafic dykes are widely distributed in the Xiaerpu granite and adjacent Carboniferous volcanic strata (Figs. 2b and 3c). The Xiaerpu dykes mainly strike 290°–310° (WNW trending) and dip at >80°. They are mostly 0.2 m to 3 m wide with some >5 m. About 5 mm chilled and baked margins against the host granitic rock are well preserved on both sides of some dykes (Fig. 3d). The rock types are dominated by dioritic porphyry and diorite with minor diabase. The dioritic porphyry (Fig. 4c) comprises phenocrysts plagioclase (10–25%) and hornblende (10–35%) set in a fine-grained (<0.02 mm) groundmass (65–75%). Cleavages and microfractures are limited to the euhedral plagioclase xenocryst interiors without passing through its overgrown rim (Fig. 4d). It is inferred that the xenocrysts and their overgrown rim were formed in original and host magmas respectively and formed by a two-stage process. The diorite has a similar mineralogy to the dioritic porphyry, but has a uniform fine-grained granular texture with plagioclase (55%-60%), hornblende (25–30%), alkali feldspar (5–10%), biotite (0–10%) and quartz (0–5%). The diabase consists of diopside (35–40%), sericitized plagioclase (50–55%), Ti-Fe oxides (5–10%) and minor hornblende (5%) and biotite (<5%). The diabase has ophitic texture with anhedral mafic minerals and Ti-Fe oxides filling in the spaces between the plagioclase laths.
A large number of dykes are found in the Liushugou area, but their distribution is dispersed compared with those of the Karamay and Xiaerpu areas. The Liushugou dykes are generally undeformed and range in width from 0.5 m to 4 m and in length from 0.1 km to 1.5 km. They mainly trend in a NE direction (Fig. 3g), with minor dykes trending to the WNW (Fig. 2d). The NE-trending dykes are diorites, and the NW trending ones are represented by dioritic porphyries. The dykes intrude Carboniferous and Ordovician sediments, the Maliya ophiolitic mélange and the Baogutu dioritic intrusion. Chilled and baked margins are not obvious between the country rocks and the dykes (Fig. 3h). Petrographically, the dykes are fine-grained diorite and dioritic porphyry and have undergone slight chloritization and sericitization. The diorite has a massive and fine to medium-grained granular texture (Fig. 4f) and consists of plagioclase (45–55%), hornblende (25–35%), quartz (<5%), orthopyroxene (0–5%) and accessory minerals such as magnetite (<3%) and apatite (<3%). The dioritic porphyry comprises 15–25% plagioclase and/or hornblende are phenocrysts, and the aphanitic matrix (75–85%) consists of plagioclase (55–65%), hornblende (30–45%) with minor quartz (<5%), pyroxene (<5%) and opaque minerals (<5%).
The Bieluagaxi dykes (Fig. 2e) are located about 1.5 km south of the Tiechanggou granite and trend ENE (60°–80°), which is parallel to the NE-trending regional faults. The dykes intrude the Devonian Kulumudi formation and small Bieluagaxi dioritic pluton and range in width from 0.2 m to 3 m, and in length from 0.1 km to 2 km. Both baked and chilled margins are found between the host pluton and the dykes. Several granular plagioclase xenocrysts were also observed on the margin of dykes against the host pluton (Fig. 3i), indicating that some magma mixing occurred between the parental magma of the Bieluagaxi pluton and the magma of the dykes. In some places, dykes have been intruded by the pluton (Fig. 3j), implying that they are coeval. The dyke swarms mostly include diorite, dioritic porphyry, with a few diabase dykes also being found. The diorite has a massive and fine-grained granular texture (Fig. 4g) with plagioclase (50–60%), hornblende (30–35%), biotite (0–5%), quartz (0–5%) and accessory minerals e.g., opaque minerals (<5%), apatite (<3%) and epidote (<3%). Dioritic porphyry displays massive and porphyritic textures (Fig. 4h). The phenocrysts (20–40%) consist of plagioclase (15–25%) and hornblende (5–15%), set in a microcrystalline matrix (60–80%) with needle-like plagioclase (45–55%), hornblende (15–25%) and minor alkal feldspar (5%). The diabases exhibit typical ophitic and massive textures and comprise diopside (35–45%), sericitised plagioclase (50–55%) and Ti-Fe oxides (5–10%) with minor hornblende (0–5%) and biotite (0–5%).
The GPS and detailed observations of dykes in central West Junggar are listed in Table S1 and Fig. 4, respectively.
4. Results
4.a Zircon U-Pb geochronology
Four samples from the dykes in West Junggar were selected for U-Pb zircon dating, and the results are listed in Table 1. The descriptions of the analytical methods used are given in Appendix 1. The zircon CL images and the concordia diagrams are presented in Figs. 5 and 6, respectively. All confidence intervals are reported at 95%.
Table 1. Whole rock major (wt. %) and trace (ppm) element of Late Carboniferous dykes in West Junggar


Figure 5. Cathodoluminescence (CL) images of zircons from Carboniferous dykes in West Junggar.

Figure 6. U-Pb Concordia diagrams showing zircon ages obtained by LA-ICP-MS for dykes in West Junggar.
Zircons in the Karamay dyke (sample KM1510N; GPS: E84°44′04″, N45°39′39″) are euhedral and prismatic with concentric oscillatory zoning and are 90–180 μm in length (Fig. 5a). The zircons have a high concentration of Th and U with Th/U ratios of 0.38–0.52. Nineteen spots form a cluster on a concordia diagram with 206Pb/238U ages ranging from 305.4 Ma to 316.4 Ma (Fig. 6a, inset) and a weighted mean 206Pb/238U age of 311.3±2.1 Ma with an MSWD of 0.74.
Zircons from Xiaerpu dyke (sample XP1505N; GPS: E84°36′18″, N45°42′35″) are transparent to pale yellow, euhedral columnar crystals. All show typical oscillatory zoning in the CL images, are 100–200 μm in length and width/length ratios ranging from 1:2 to 1:3 (Fig. 5b). Zircon grains have Th contents ranging from 204 to 522 ppm and U contents ranging from 242 to 930 ppm with relatively high Th/U ratios of 0.35–0.85. Fourteen spots yield a weighted mean 206Pb/238U age of 309.7±2.4 Ma (Fig. 6b, inset) with MSWD of 1.08.
Zircons from the Bieluagaxi dyke (sample BLG1505N; GPS: E84°24′19″, N46°02′09″) are prismatic and euhedral crystals with rhythmic oscillatory zoning and are 85–180 μm in length (Fig. 5c). The zircons have high U and Th contents with relatively high Th/U ratios (0.55–0.75). Thirteen spots yield a weighted mean 206Pb/238U age of 324.3±3.6 Ma (Fig. 5c) with MSWD of 0.94.
Zircons from Liushugou dyke (sample LSG1501N; GPS: E84°17′40″, N45°30′59″) show internal oscillatory zonation. They are 100–200 μm in length and are mostly subhedral-euhedral prismatic crystals (Fig. 5h). They have high Th/U contents of 0.55–1.18, indicating a magmatic origin. Seventeen spots of sample LSG1501N yield a weighted mean 206Pb/238U age of 313.9±3.2 Ma with an MSWD of 0.31 (Fig. 6h).
4.b Whole-rock geochemistry
Analytical methods are described in Appendix 1 and the whole-rock elemental data for the dykes in the West Junggar area are shown in Table 2 and S4. A few samples have relatively high LOI values of 3.00–5.37 wt.%, however, most samples have LOI values of 1.88–2.99 wt.%, (Table 2), supporting a low degree of alteration or low-grade metamorphism. The major oxides and trace elements do not display any obvious correlations with LOI values (Table S2 and Fig. S1), further suggesting that their abundances are unlikely to have been modified by the low-grade metamorphism or alteration.
Table 2. Zircon U-Pb LA-ICP-MS geochronological data of Late Carboniferous dykes in West Junggar

The dykes in West Junggar have variable low – intermediate SiO2 (52.3 – 60.9 wt.%), moderate – high Al2O3, and along with relatively low TiO2 and FeOT (Table 2). They have low Nb/Y ratios of 0.13–0.31 (except sample BLG1502 with Nb/Y = 0.85) and plot in the fields of andesite and andesite/basalt on the Nb/Y vs. Zr/TiO2 diagrams (Fig. 7a). The dykes have moderate total alkali content (ALK = Na2O+K2O) ranging from 3.69 and 5.72 wt.% with Na2O/K2O ratios of 2.14–7.23 and are classified as gabbroic diorite (Liushugou and Bieluagaxi dykes) and diorite (Karamay and Xiaerpu dykes) in the ALK vs. SiO2 diagram (not shown). Most of the dykes show medium-K calc-alkaline and minor with high-K calc-alkaline and arc tholeiite characteristics (Fig. 7b). They possess variable MgO contents between 2.6 wt.% and 8.4 wt.% with Mg# of 51–69 (Mg# = 100*Mg2+/(Mg2++Fe2+)) and low ratios of (La/Yb) N (3.6 – 14.0), consistent with many of the features of HMAs (McCarron & Smellie, Reference McCarron and Smellie1998; Kelemen et al. Reference Kelemen, Yogodzinski and Scholl2003; Kamei et al. Reference Kamei, Owada, Nagao and Shiraki2004).

Figure 7. (a) Zr/TiO2 versus Nb/Y and (b) K2O versus SiO2 (Gill, Reference Gill1981) diagrams. In (a), the data for sanukitic HMAs in the Setouchi volcanic belt are from Shimoda et al. (Reference Shimoda, Tatsumi, Nohda, Ishizaka and Jahn1998), Tatsumi (Reference Tatsumi2001) and references therein.
Based on the distribution according to their orientation, petrology and geochronology of dykes in West Junggar, they can be divided into three groups (Table S1): the Early stage is represented by ENE-trending Bieluagaxi dykes, which were generated at 324 Ma and include diorite, dioritic porphyrite, with minor diabase; the Late 1 stage contains WNW-trending dykes formed between 314 Ma and 310 Ma, including dioritic porphyrites in Karamay, Xiaerpu and Liushugou areas; the last stage is referred as Late 2 stage marked by relatively younger NE-trending diorite with minor diabase dykes (<310 Ma) in Karamay and Liushugou areas.
In view of the similar geochemical characteristics of the Late 1 and 2 stage dykes (Figs. 6–8, Table 2 and S4), we divide dykes in West Junggar into two groups. Karamay, Xiaerpu and Liushugou dykes (Group 1: Late 1 and 2 stage) and Bieluagaxi dykes (Group 2: Early stage).

Figure 8. (a) MgO versus SiO2 (McCarro and Smellie, 1998), (b) Sr/Y versus Y diagrams (Defant and Drummond, Reference Defant and Drummond1990), (c) TiO2 versus MgO/(MgO+FeOT) (Kamei et al. Reference Kamei, Owada, Nagao and Shiraki2004) and (d) LaN/YbN versus YbN (Kamei et al. Reference Kamei, Owada, Nagao and Shiraki2004) diagrams. In (a), the field of metabasaltic melts is from Rapp and Watson (Reference Rapp and Watson1995), and the fields of the subducted oceanic crust-derived, the thickened lower crust-derived adakites are from Wang et al. (Reference Wang, Li, Chung, Wyman, Sun, Zhao, Zhu and Qiu2011). Sanukitoid data of the Setouchi volcanic belt and Karamay-Baogutu area are from Tatsumi et al. (Reference Tatsumi, Shukuno, Sato, Shibata and Yoshikawa2003), Tang et al. (Reference Tang, Wang, Wyman, Li, Xu and Zhao2012a), Ma et al. (Reference Ma, Xiao, Windley, Zhao, Han, Zhang, Luo and Li2012) and Yin et al. (Reference Yin, Yuan, Sun, Long, Zhao, Wong, Geng and Cai2010, Reference Yin, Long, Yuan, Sun, Zhao and Geng2013), respectively in (a)–(d).
Group 1 samples have relatively low – moderate rare earth element (REEs) concentrations (ΣREE 40 – 65 ppm). They display coherent REE patterns that are enriched in the LREEs [(La/Yb) N = 3.6 – 8.9] with depleted HREE patterns [(Gd/Yb) N = .50 – 1.90] and slightly negative to positive Eu anomalies (0.9 – 1.1) (Table 2; Fig. 10a, c and g). They have variable Cr (26 – 276 ppm) and Ni (29 – 159 ppm), high Sr (564 – 748 ppm), Sr/Y ratios of 40.5 – 82.9 (Yin et al. Reference Yin, Yuan, Sun, Long, Zhao, Wong, Geng and Cai2010, Reference Yin, Long, Yuan, Sun, Zhao and Geng2013; Ma et al. Reference Ma, Xiao, Windley, Zhao, Han, Zhang, Luo and Li2012; Tang et al. Reference Tang, Wang, Wyman, Li, Xu and Zhao2012a) and Ba (376 – 1163 ppm) with depletion in Yb (0.86 – 1.67 ppm) and Y (9.03 – 16.8 ppm) (Table 2).

Figure 9. Harker diagrams (a-f), Th/Nd vs. Th (g) (Schiano et al. Reference Schiano, Monzier, Eissen, Martin and Koga2010) and La/Sm vs. La (h) (Treuil & Joron, Reference Treuil and Joron1975) diagrams for Carboniferous dykes in West Junggar. All the other data refers to Appendix 1 and the data on dykes in West Junggar are from He (Reference He2012), Chen (Reference Chen2015), He et al. (Reference He, Xiao, Wang, Gao, Yang and Yan2015), Ma et al. (Reference Ma, Xiao, Windley, Zhao, Han, Zhang, Luo and Li2012), Tang et al. (Reference Tang, Wang, Wyman, Li, Xu and Zhao2012a), Yin et al. (Reference Yin, Yuan, Sun, Long, Zhao, Wong, Geng and Cai2010, Reference Yin, Long, Yuan, Sun, Zhao and Geng2013, Reference Yin, Chen, Xiao, Yuan, Sun, Tang, Yu, Long, Cai, Geng, Zhang and Liu2015a, Reference Yin, Chen, Yuan, Yu, Xiao, Li and Sun2015b) and Zhang and Zou, (Reference Zhang and Zou2013). Trace plots for the discrimination of partial melting, fractional crystallisation and magma mixing in (g) are from (Schiano et al. Reference Schiano, Monzier, Eissen, Martin and Koga2010).
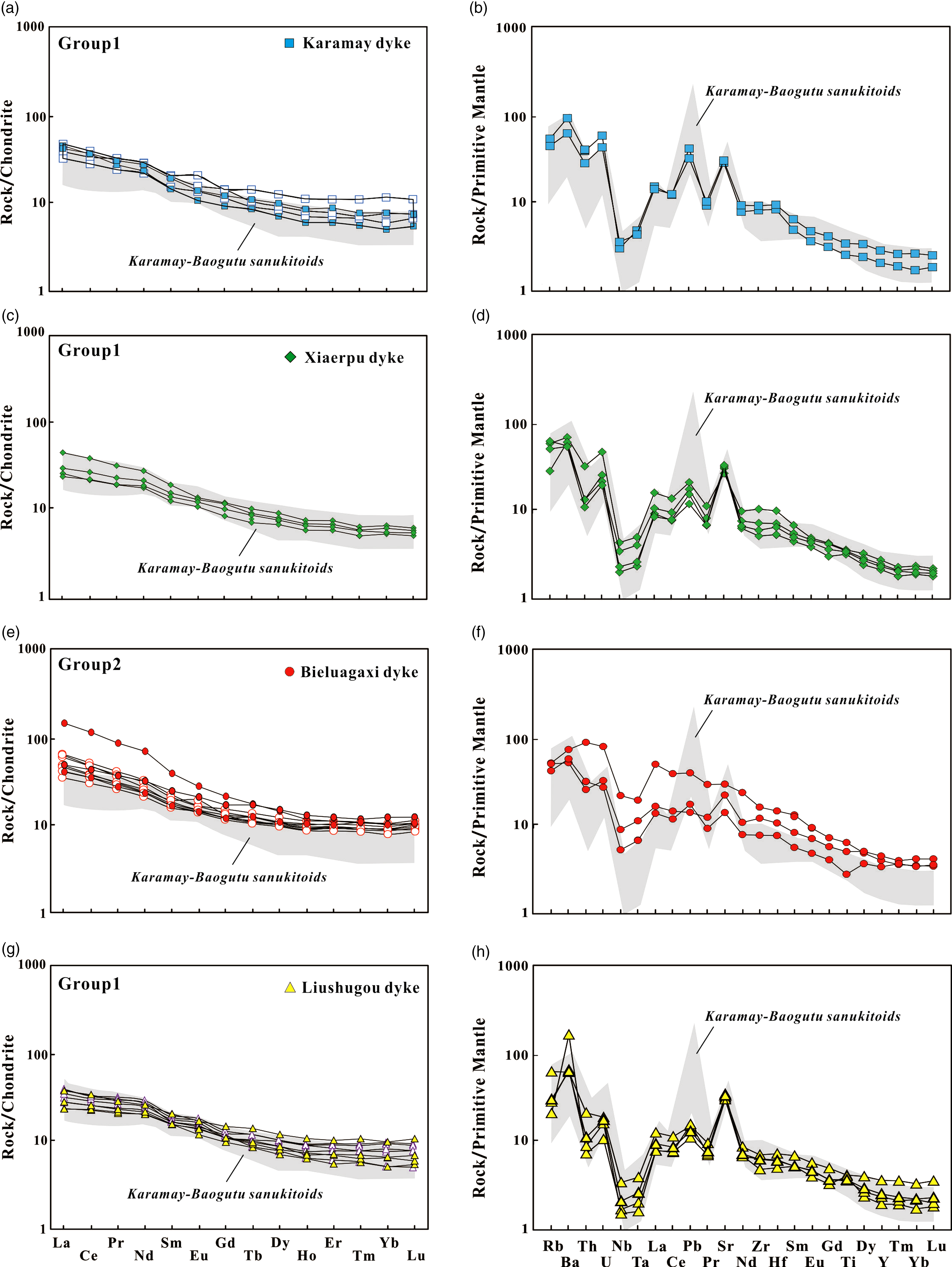
Figure 10. Chondrite-normalised rare earth element diagram (a, c, e and g) and primitive mantle-normalised trace-element variation diagram (b, d, f and h) of dykes in West Junggar. Chondrite, Primitive mantle, MORB and OIB data are from Sun and McDonough (Reference Sun and McDonough1989). The Karamay-Baogutu area is from Tang et al. (Reference Tang, Wang, Wyman, Li, Xu and Zhao2012a), Ma et al. (Reference Ma, Xiao, Windley, Zhao, Han, Zhang, Luo and Li2012) and Yin et al. (Reference Yin, Yuan, Sun, Long, Zhao, Wong, Geng and Cai2010, Reference Yin, Long, Yuan, Sun, Zhao and Geng2013).
Group 2 rocks are also enriched in LREE relative to HREE with (La/Yb) N ratios of 3.94–14.03 and (Gd/Yb) N ratios of 1.19–2.06 (Table 2; Fig. 10e) along with weak negative to weak positive Eu anomalies (Group 1: Eu*/Eu = 0.88–1.07; Group 2 of 0.91–1.01). Compared with Group 1 samples, they have similar Cr and Ni contents but lower Sr (294–618 ppm), Ba (358–503 ppm) and Sr/Y ratios of 19.1–33.9 (Fig. 8b). On primitive mantle-normalised multi-element diagrams (Fig. 10), Group 1 as well as being enriched in Light Rare Earth Element (LREE) and Large Ion Lithophile Element (LILE) are depleted in High Field Strength Element (HFSE, e.g., Nb and Ta) (Fig. 10b, d and h). Group 2 samples have a similar pattern to Group 1 rocks but with relatively lower Nb and Ta, and higher Sr and Ba (Fig. 10f).
In summary, all studied dykes resemble HMAs and are characterised by intermediate SiO2, high MgO, high Cr and Ni contents, marked Nb and Ta depletions, high Sr, Ba and highly fractionated REE (Martin et al. Reference Martin, Smithies, Rapp, Moyen and Champion2005; Yin et al. Reference Yin, Yuan, Sun, Long, Zhao, Wong, Geng and Cai2010).
4.c Sr-Nd isotopes
Analytical methods for whole-rock Sr-Nd analyses are given in Appendix 1. Eight representative dyke samples were analysed for Sr and Nd isotopes and these results are listed in Table 3. All measured 87Sr/86Sr ratios and εNd values have been corrected to the corresponding ages of dykes (Table 3; Fig. 11a). The (87Sr/86Sr) i ratios of the Karamay dykes range from 0.7035 to 0.7039, with an εNd(t) value of + 7.8 and the Nd model age (TDM) of 0.52 Ga. The Xiaerpu dykes exhibit εNd(t) values of +4.5 – +7.0, (87Sr/86Sr) i ratios of 0.7037–0.7039 and TDM of 0.58 – 0.83 Ga. The Bieluagaxi dykes have (87Sr/86Sr) i ratios of 0.7041 to 0.7044 and positive εNd(t) values ranging from +4.4 to +5.7 with TDM of 0.62–0.69 Ga. The Liushugou dykes have εNd(t) values between +5.2 and +7.4, (87Sr/86Sr) i ratios ranging from 0.7038 to 0.7044 and TDM from 0.53 to 0.77 Ga. Thus, all studied dykes in West Junggar have positive εNd(t) values (+4.4 to +7.8), low (87Sr/86Sr) i ratios (0.7035 – 0.7044) and young Nd model ages (TDM = 0.52 – 0.83 Ga).
Table 3. Sr-Nd isotopic data of the Late Carboniferous dykes in West Junggar

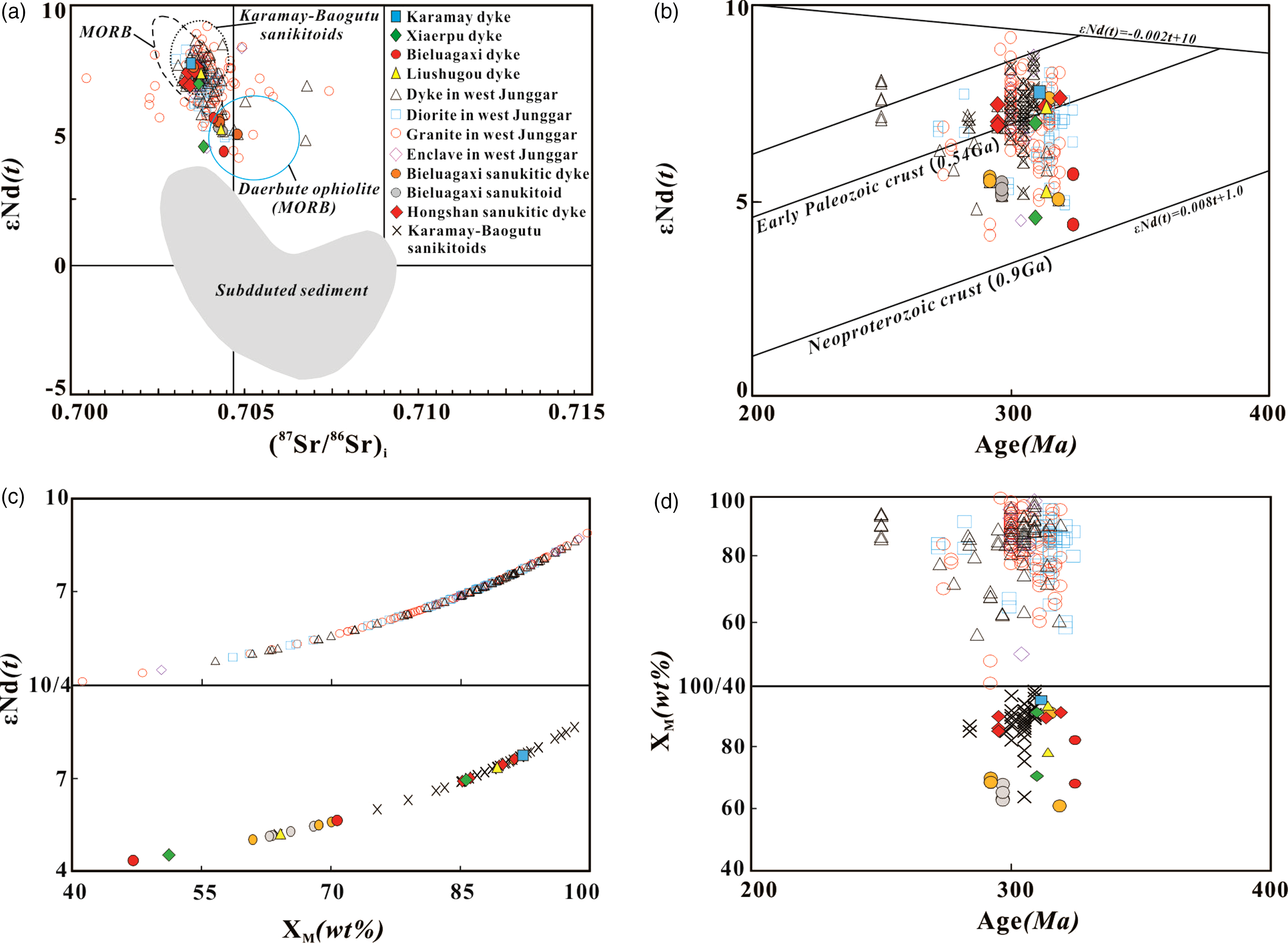
Figure 11. εNd(t) versus (87Sr/86Sr) i (a), εNd(t) versus age (b), estimated proportion of mantle-derived materials in magmatic rocks based on Nd isotope two-end member mixing calculation (c) and estimated mantle proportion versus age of the igneous rocks in Central West Junggar (d). The Sr-Nd isotopic data of granite and diorite in West Junggar are from Geng et al. (Reference Geng, Sun, Yuan, Xiao, Xian, Zhao, Zhang, Wong and Wu2009), Tang et al. (Reference Tang, Wang, Wyman, Li, Zhao and Yang2012b), Xu et al. (Reference Xu, Ji, Zhao, Gong, Zhou, He, Zhong, Wang and Griffiths2013), Gao et al. (Reference Gao, Xiao, Pirajno, Wang, He, Yang and Yan2014), Yin et al. (Reference Yin, Chen, Xiao, Yuan, Sun, Tang, Yu, Long, Cai, Geng, Zhang and Liu2015a), Li et al. (Reference Li, He and Fan2015), Liu et al. (Reference Liu, Han, Chen, Ren, Zheng, Wang and Feng2017) and Zheng et al. (Reference Zheng, Han, Wang, Liu and Feng2020); the Sr-Nd isotopic data of dykes in West Junggar are from Yin et al. (Reference Yin, Yuan, Sun, Long, Zhao, Wong, Geng and Cai2010, 2012,Reference Yin, Yuan, Sun, Long, Qiu, Wang, Ren and Guan2012, Reference Yin, Long, Yuan, Sun, Zhao and Geng2013, Reference Yin, Chen, Xiao, Yuan, Sun, Tang, Yu, Long, Cai, Geng, Zhang and Liu2015a, Reference Yin, Chen, Yuan, Yu, Xiao, Li and Sun2015b), Tang et al. (Reference Tang, Wang, Wyman, Li, Zhao, Jia and Jiang2010, Reference Tang, Wang, Wyman, Li, Xu and Zhao2012a, Reference Tang, Wang, Wyman, Li, Zhao and Yang2012b), Ma et al. (Reference Ma, Xiao, Windley, Zhao, Han, Zhang, Luo and Li2012), Xu et al. (Reference Xu, Ji, Zhao, Gong, Zhou, He, Zhong, Wang and Griffiths2013), He et al. (Reference He, Xiao, Wang, Gao, Yang and Yan2015), Li et al. (Reference Li, He and Fan2015), Zhan et al. (Reference Zhan, Hou, Hari and Shu2015), Duan et al. (Reference Duan, Li, Zhi, Yang and Gao2019) and Ma et al. (Reference Ma, Chen, Xu, Ma, Han, Ding and Wang2020). The Sanukitoid data of the Karamay-Baogutu area are from Tang et al. (Reference Tang, Wang, Wyman, Li, Xu and Zhao2012a), Ma et al. (Reference Ma, Xiao, Windley, Zhao, Han, Zhang, Luo and Li2012) and Yin et al. (Reference Yin, Yuan, Sun, Long, Zhao, Wong, Geng and Cai2010, Reference Yin, Long, Yuan, Sun, Zhao and Geng2013). In (d) and (e), the number represents the fractionated number. In (f)–(h), F is fractionated number. Partition coefficients are from Rollinson (Reference Rollinson1993), Green and Pearson (Reference Green and Pearson1986), Mahood and Hildreth (Reference Mahood and Hildreth1983) and Yurimoto et al. (Reference Yurimoto, Duke, Papike and Shearer1990). Abbreviations: Ol = olivine, Opx = orthopyroxene, Cpx = clinopyroxene, Hb = hornblende, Ap = apatite, Mon = monazite, Allan = allanite.
5. Discussion
5.a Age correlation between dykes and host rocks
The zircons from the dykes in West Junggar have high Th/U ratios (Table 1) with oscillatory zoning shown by the CL images (Fig. 5), suggesting magmatic origins. However, most of the zircons in the dykes show typical alternating light and dark oscillatory zoning to those in the felsic magmatic rocks. The ages of zircons from the dykes are similar to the host granitic rocks, and so it is possible that the dated zircons in dykes were captured by the Carboniferous granitic host rocks. This conclusion is supported by the geological intercalated relationship between the dykes and the host granitic rocks. Both the dykes and the host granitic rocks underwent plastic interactions, indicating that the host rocks were still semi-crystalline, and even hot, when the dykes were emplaced (Ma et al. Reference Ma, Xiao, Windley, Zhao, Han, Zhang, Luo and Li2012). Therefore, based on the weighted average 206Pb/238U age of zircons (Karamay dykes, 311 Ma; Xiaerpu dykes, 310 Ma; Bieluagaxi dykes, 324 Ma; Liushugou dykes, 314 Ma), and the field relationship between the dykes and the host granitic rocks, the dykes were formed almost simultaneously or slightly later than the host granitic rocks in the Late Carboniferous.
All studied dykes intrude into their corresponding host plutons except for the Liushugou dykes, which intrude into Devonian to Carboniferous strata (Fig. 2d). The Late Carboniferous – Early Permian host rocks (e.g., Karamay, Xiaerpu, Hongshan and Bieluagaxi granitoid complexes) in West Junggar are well-dated (Table S3) and consist of syenogranite + alkali-feldspar granite ± monzogranite ± granodiorite ± diorite. These granitoids have emplacement ages between 276 Ma and 319 Ma (Table S3). Based on reported data (Chen & Jahn, Reference Chen and Jahn2004; Han et al. Reference Han, Ji, Song, Chen and Zhang2006; Geng et al, Reference Geng, Sun, Yuan, Xiao, Xian, Zhao, Zhang, Wong and Wu2009; Tang et al., Reference Tang, Wang, Wyman, Li, Zhao, Jia and Jiang2010, Reference Tang, Wang, Wyman, Li, Zhao and Yang2012b; Yin et al. Reference Yin, Yuan, Sun, Long, Zhao, Wong, Geng and Cai2010, Reference Yin, Yuan, Sun, Long, Qiu, Wang, Ren and Guan2012, Reference Yin, Long, Yuan, Sun, Zhao and Geng2013, Reference Yin, Chen, Xiao, Yuan, Sun, Tang, Yu, Long, Cai, Geng, Zhang and Liu2015a, Reference Yin, Chen, Yuan, Yu, Xiao, Li and Sun2015b; Li et al. Reference Li, Li, Li, Wang and Gao2013, Reference Li, He and Fan2015; Gao et al. Reference Gao, Xiao, Pirajno, Wang, He, Yang and Yan2014; He et al. Reference He, Xiao, Wang, Gao, Yang and Yan2015; Duan et al. Reference Duan, Li, Zhi, Yang and Gao2019; Ma et al. Reference Ma, Chen, Xu, Ma, Han, Ding and Wang2020), the Karamay dykes and host pluton have ages between 298 Ma and 317 Ma, and between 276 Ma and 319 Ma, respectively. The emplacement ages of Xierpu granitoids and dykes range from 298 Ma to 311 Ma and from 299 Ma to 310 Ma, respectively. Bieluagaxi granitoids and dykes have the ages of 299 Ma – 319 Ma and 292 Ma – 324 Ma. Liushugou dykes, intruding into the C1 strata, have similar age to those of dykes (314 Ma– 321 Ma, Tang et al. Reference Tang, Wang, Wyman, Li, Zhao, Jia and Jiang2010; An et al. Reference An, Zhu, Lehmann, Zheng and Qiang2022a) and plutons (310 Ma – 315Ma) from Baogutu area.
The dykes mostly extend linearly (Fig. 3a, c, e) but some of them are curved or locally deformed (Fig. 3b, d, h). An extensional stress regime is evident due to the irregular jagged contact boundary between dykes and country rocks during the intrusion of these dykes into the pre-existing fractures (Fig. 3b, d, f, h). Thus, based on field observations and petrographic analysis along with geochronological data, we propose that all the dykes were formed after the intrusion of host granitoids, whereas the interval between them is short. When the dykes intruded into the host granitoids, the latter has not been completely cooled and consolidated. This is supported by the proposition that the emplacement interval between the Karamay granitoid and the dykes may have been about one million years (Ma et al. Reference Ma, Xiao, Windley, Zhao, Han, Zhang, Luo and Li2012).
5.b Petrogenesis of HMA dykes
The studied dykes display similar geochemical features to HMAs with variable SiO2, MgO and high Mg# (Fig. 9b) (Kamei et al. Reference Kamei, Owada, Nagao and Shiraki2004). HMAs are generally divided into four types, namely boninite, bajaite, high-Mg adakite and sanukite (Kamei et al. Reference Kamei, Owada, Nagao and Shiraki2004). Boninites are characterised by high MgO (>8%) and very low TiO2 (< 0.5%) (McCarron & Smellie, Reference McCarron and Smellie1998), which is obviously different from the studied samples. The bajaites have high Sr (>000 ppm) and Ba (>1000 ppm) concentrations, which are also not consistent with our samples (Sr from 294 ppm to 748 ppm; Ba of 358 ppm – 642 ppm with only one sample of 1163 ppm). High-Mg adakites show relatively high SiO2 (>56%), MgO (< 3%), high Mg# values, Sr (>400 ppm), Sr/Y (>40) and (La/Yb) N (>10) ratios with low Y (<18 ppm) and Yb (<1.8 ppm) (Defant & Drummond, Reference Defant and Drummond1990). Sankitoids are characterised by high MgO (generally >5%), Mg# (>45) values, high Cr and Ni (both >100 ppm), relatively low Sr and Ba concentrations with low Sr/Y (<40) and (La/Yb) N (<10) ratios (Kelemen, Reference Kelemen1995; Tatsumi, Reference Tatsumi2006). In the classification diagrams for HMAs, nearly all the dyke samples plot in the field of sanukite (Fig. 8c and d).
Group 1 dykes show relative enrichment of LREEs with fractionated HREE patterns (Fig. 10e), high Sr (564 ppm–748 ppm) and Sr/Y ratios (40.47–82.85) with low Y (9.03 ppm–16.8 ppm) and Yb (0.86 ppm–1.67 ppm) concentrations (Table 2). Group 2 samples have similar enrichment of LREEs and fractionated HREE patterns (Fig. 10e), with relatively lower Sr (294 ppm – 618 ppm) and Ba (358 ppm – 503 ppm), and higher Ni (94. 3 ppm – 135 ppm) and Cr (133 ppm – 402 ppm) concentrations (Table 2).
Using data from He (Reference He2012) and Chen (Reference Chen2015), along with our new data, Group 2 dykes have higher MgO contents (4.42–8.41, mean value of 7.09, Table 1 and S4) than those of Group 1 (2.56–6.13, mean value of 4.61, Table 1 and S4), and majority of both groups plot in the high-Mg andesite area with a few samples in the Karamay – Baogutu sanukitoids area (Fig. 9b) except one sample from Karamay area (H8552-1, Table S4). Compared with geochemical characteristics of high-Mg adakites and sanukitoids, Group 1 is similar to Karamay-Baogutu sanukitoids [these are widespread in Karamay – Baogutu area in central West Junggar and show similar geochemical features to sanukitic rocks with low (La/Yb) N ratios but classify as adakites field with high Sr/Y ratios, Yin et al. (Reference Yin, Yuan, Sun, Long, Zhao, Wong, Geng and Cai2010, Reference Yin, Long, Yuan, Sun, Zhao and Geng2013); Ma et al. (Reference Ma, Xiao, Windley, Zhao, Han, Zhang, Luo and Li2012)], and Group 2 shows similarities to sanukitoids.
5.b.1 Crustal contamination and fractional crystallisation
Despite some compositional differences observed among various dykes in both groups of samples, they share relatively similar low initial (87Sr/86Sr)i ratios and high positive εNd(t) values (Table 3; Fig. 11a). The Nb/La and (87Sr/86Sr)i ratios are essentially constant with increasing Mg# and SiO2 (Fig. 12a and b), which rules out significant crustal contamination during magma ascent and emplacement. This interpretation is also consistent with the lack of significant correlation between Nb/La and Sm/Nd (Figure not shown).

Figure 12. Nb/La versus Mg# (a) (He et al. Reference He, Xiao, Wei and Ni2009), (87Sr/86Sr)i versus SiO2 (b), U/Yb versus Y (c) (Grimes et al. Reference Grimes, John, Kelemen, Mazdab, Wooden, Cheadle, Hanghoj and Schwartz2007), modelled Ni versus Cr (d), modelled (La/Yb) N versus La (e) and the rare earth elements changes with different degree of apatite fractionated of Group 1 Liushugou and Xiaerpu dykes in Group 1 (f), zircon fractionated of Group 1 Karamay dykes (g), and monazite fractionated of Group 2 Bieluagaxi dykes (h).
The negative correlations of TiO2, FeOT, MgO and CaO with SiO2, for the two groups of dykes (Fig. 9a, b, d and e), indicate fractional crystallisation of olivine and pyroxene. There is no obvious correlation between Al2O3, total alkalis and silica (Fig. 9f). The trace element variation in these dykes, when combined with data from other reported dykes in West Junggar, also suggests that fractional crystallisation of olivine and orthopyroxene has taken place (Fig. 12d), which is consistent with the presence of hornblende + biotite as the dominant mafic minerals/phenocrysts in the rocks (Fig. 4a, c, e, f and h). The Cr and Ni abundances of Group 1 dykes can be quantitatively explained by the 0–18% fractionation of 95% orthopyroxene and 5% olivine (Fig. 12d) starting from the assumed parent magma composition represented by the relatively least fractionated sample H8747-5 from Group 1 samples. The quantitative simulation of the Cr and Ni abundances from Group 2 samples could be derived from 0 to 10% fractionation of 75% orthopyroxene and 25% olivine starting from the assumed parent magma composition represented by the least fractionated sample BLG1505h (Fig. 12d). The noticeable positive Sr anomalies and negligible negative Eu anomalies demonstrate that plagioclase was not a major fractionating phase in either group (Fig. 10). The positive linear correlation in the (La/Yb) N ratios vs. La diagram (Fig. 12e) for the Liushugou and Xiaerpu dykes suggests that the 0–0.02% fractional crystallisation of apatite could explain the composition of Group 1 samples (Fig. 12e, f). Modelling suggests that the Karamay samples in Group 1 experienced 0–0.02% fractional crystallisation of zircon (Fig. 12e, and g). Group 2 samples experienced fractional crystallisation of 0–0.05% monazite starting from the assumed parent magma composition represented by the least fractionated sample BLG1505h.
5.b.2 Petrogenesis of Group 1 dykes
The Group 1 dykes in West Junggar also have high Mg# values, Ni and Cr concentrations, indicating that they are likely to be melts derived from the mantle (Rapp & Watson, Reference Rapp and Watson1995). They have relatively low (87Sr/86Sr)i (0.7035–0.7044) and slightly high εNd(t) values (+4.5–+7.8), which are similar to the granitoids and dykes, which are derived from partial melting of the basement (i.e., subducted and trapped juvenile oceanic crust, Xu et al. Reference Xu, Ji, Zhao, Gong, Zhou, He, Zhong, Wang and Griffiths2013; Gao et al. Reference Gao, Xiao, Pirajno, Wang, He, Yang and Yan2014; Zhang et al. Reference Zhang, Wang, Polat, Zhu, Shen, Chen, Chen, Guo, Wu and Liu2018; Zheng et al. Reference Zheng, Han, Wang, Liu and Feng2020) in West Junggar (Fig. 11a; Table S5). The Group 1 dykes have high Th/La and low Nb/Th ratios that plot in the compositional spectrum of forearc basalts, indicating an oceanic slab-related origin (Fig. 14e) and this is also consistent with high Th/Yb ratios relative to Mid Ocean Ridge Basalt (MORB) (Fig. 14f). All of these features indicate an oceanic crust source and their young Nd model ages (T DM ) prove that their source is juvenile.

Figure 13. TiO2 vs. SiO2 (a), Mg# vs. SiO2 (b), Th/Ce vs. SiO2 (c) and Ni vs. SiO2 (d) diagrams. Fields of subducted oceanic crust-derived adakites, thick lower crust-derived adakitic-like rocks and delaminated lower crust-derived adakitic-like rocks are after Wang et al. (Reference Wang, Xu, Jian, Bao, Zhao, Li, Xiong and Ma2006).

Figure 14. Th/Yb versus Ba/La (a) (Woodhead et al. Reference Woodhead, Hergt, Davidson and Eggins2001), and Th/Yb versus Th/Sm (b) (Liu et al. Reference Liu, Ma, Tang, Dong, Xu, Zhao, Sun and Huang2021), Th/La versus Th (c) (Plank and Langmuir, Reference Plank and Langmuir1998), Th/La versus Sm/La (d) (Plank, Reference Plank2005), Normal-MORB (Sun & McDonough, Reference Sun and McDonough1989) normalised plots of (Th/La) N-MORB versus Nb/Th N-MORB (e) (Liu et al. Reference Liu, Xu, Castillo, Xiao, Shi, Feng and Guo2014) and Th/Yb versus Ta/Yb (f) (Pearce, Reference Pearce1982) diagrams of the Carboniferous dykes in West Junggar. The trace-element data are from He, Reference He2012, Ma et al. Reference Ma, Xiao, Windley, Zhao, Han, Zhang, Luo and Li2012, Tang et al. Reference Tang, Wang, Wyman, Li, Xu and Zhao2012a, Chen, Reference Chen2015, He et al. Reference He, Xiao, Wang, Gao, Yang and Yan2015 and Yin et al. Reference Yin, Yuan, Sun, Long, Zhao, Wong, Geng and Cai2010, Reference Yin, Yuan, Sun, Long, Qiu, Wang, Ren and Guan2012, Reference Yin, Long, Yuan, Sun, Zhao and Geng2013, Reference Yin, Chen, Xiao, Yuan, Sun, Tang, Yu, Long, Cai, Geng, Zhang and Liu2015a, Reference Yin, Chen, Yuan, Yu, Xiao, Li and Sun2015b. In (a), the sanukitic data of Setouchi volcanic belt are from Tatsumi (2003). In (d)–(f), the Sanukitoid data of the Karamay-Baogutu area are from Tang et al. (Reference Tang, Wang, Wyman, Li, Xu and Zhao2012a), Ma et al. (Reference Ma, Xiao, Windley, Zhao, Han, Zhang, Luo and Li2012) and Yin et al. (Reference Yin, Yuan, Sun, Long, Zhao, Wong, Geng and Cai2010, Reference Yin, Long, Yuan, Sun, Zhao and Geng2013). The data of EMORB, N-MORB and Mantle are from Sun and McDonough (Reference Sun and McDonough1989), and the GLOSS is referred by Plank and Langmuir (Reference Plank and Langmuir1998).
High magnesium magmas can be generated by the partial melting of hydrous peridotite mantles (Hirose, Reference Hirose1997), particularly when hydrous felsic liquids from the subducting oceanic slab or sediment-derived melts trigger partial melting (Mukasa et al. Reference Mukasa, Blatter and Andronikov2007). In a subduction zone, Sr and Ba are mobile in aqueous fluids, while LREE and Th are preferentially transferred from the slab when sediment-derived melts are involved. Therefore, ratios such as Ba/La, Th/La, Th/Yb and Sr/Yb can be used to assess the influence of aqueous fluids and sediment-derived melts (Johnson & Plank, Reference Johnson and Plank1999). Apart from Liushugou dykes with variable Ba/La ratios suggesting that their generation was related to the oceanic-slab-derived fluid, Group 1 dykes have high Th/Yb but low Ba/La ratios (e.g., Fig. 14a), indicating that sediment-derived melt plays a dominant role in the formation of most dykes in West Junggar. However, the magmatic zircons in both groups of dykes have moderate U/Yb ratios and Y contents (Fig. 12c), which are consistent with both continental zircon and ocean crust zircon (Grimes et al. Reference Grimes, John, Kelemen, Mazdab, Wooden, Cheadle, Hanghoj and Schwartz2007). In Fig. 14b, c and d, the samples plot on a trend between GLOSS or sediment and MORB, indicating interaction between peridotite mantle and sediment-derived melt.
The retention of zircons in the sedimentary residue would result in significant depletion of Zr and Hf in the resultant magmas (Woodland et al. Reference Woodland, Bulatov, Brey, Girnis, Höfer and Gerdes2018). Both groups of samples do not show negative Zr-Hf anomalies on primitive mantle-normalised diagrams (Fig. 10b, d, f and h), indicating no or few residual zircons and a high degree of sediments melting. Furthermore, the presence of broad or weak zoning in the CL images of both group dykes indicates that the zircons in these rocks crystallised from high-temperature melts (Fig. 5; Wu & Zheng., Reference Wu and Zheng2004), which is also confirmed by the crystallisation temperatures (Table S6) of the magmatic zircons (estimated by using the Ti-in-zircon thermometry, Ferry & Watson, Reference Ferry and Watson2007). Karamay, Xiaerpu and Liushugou dykes (Group 1) have crystallisation temperatures of 789 °C–940 °C, 804 °C–1006 °C and 772 °C–1112 °C.
Several models have been proposed to explain the relatively high Sr/Y ratios and Mg# values of the HMAs, including (1) magma mixing with subsequent contamination (Guo et al. Reference Guo, Nakamuru, Fan, Kobayoshi and Li2007); (2) fractional crystallisation of mantle-derived basaltic magma (Li et al. Reference Li, Zhao, Zhou, Ma, Souza and Vasconcelos2009, Reference Li, Li, Li, Wang and Gao2013); and (3) melt-mantle interaction (Chung et al. Reference Chung, Liu, Ji, Chu, Lee, Wen, Lo, Lee, Qian and Zhang2003; Gao et al. 2004; Martin et al. Reference Martin, Smithies, Rapp, Moyen and Champion2005). Group 1 dykes have relatively similar low initial (87Sr/86Sr) i ratios and high positive εNd(t) values (Table 3; Fig. 11a), which precludes the possibility of crustal contamination. Geochemical modelling of batch partial melting, fractional crystallisation and magma mixing can be used to investigate the processes that exert an influence on the trace-element variations of cogenetic magmas (Schiano et al. Reference Schiano, Monzier, Eissen, Martin and Koga2010). Modelling of partial melting and fractional crystallisation would produce a straight line with a slope and a horizontal line, respectively, in plots of two incompatible elements with different partition coefficients (e.g., Th/Nd vs. Th, La/Sm vs. La; Fig. 9g and h) (Treuil and Joron, Reference Treuil and Joron1975; Schiano et al. Reference Schiano, Monzier, Eissen, Martin and Koga2010). These plots of the Group 1 dykes show that they could have been generated by partial melting rather than fractional crystallisation or magma mixing (Fig. 9g and h). Hence, the model (1) and (2) can be ruled out. This conclusion is also supported by geological and geochemical observations, including (1) the general absence of mafic magmatism in central West Junggar during the Late Carboniferous – Early Permian. Therefore, fractional crystallisation cannot account for the widespread coeval intermediate to felsic magmas; (2) the lack of disequilibrium texture of phenocrysts and the presence of compositional ortho-zonal structure in plagioclase phenocrysts in the Group 1 dykes suggest that there has not been extensive magma mixing; (3) The Mg# of these high-Mg rocks are comparable to those of crust-derived melts that underwent assimilation with peridotite in the mantle (Fig. 13b; Rapp et al. Reference Rapp, Shimizu, Norman and Applegate1999). (4) The dykes coexist with Nb-enriched-like rocks in central West Junggar, which are generally considered to be produced by the melting of mantle metasomatized by slab-derived melts (Yin et al. Reference Yin, Long, Yuan, Sun, Zhao and Geng2013).
There are three mechanisms for melt-mantle interaction between peridotite mantle and the (1) subducting oceanic slab-derived melt (e.g., Defant & Drummond, Reference Defant and Drummond1990); (2) delaminated lower crust-derived melt (e.g., Gao et al. 2004); or (3) thickened lower crust-derived melt (e.g., Wang et al. Reference Wang, Xu, Jian, Bao, Zhao, Li, Xiong and Ma2006). The subducting slab-derived melt and subsequent interaction with mantle is preferred based on the following lines of evidence: (1) The major and trace-element compositions of the West Junggar dykes fit well within the fields of subducting oceanic slab (Figs. 8a, and 12). They have high MgO, Mg#, Ni and Cr contents, which resemble those of subducting oceanic slab-derived adakitic-like rocks (Defant & Drummond, Reference Defant and Drummond1990). They have low TiO2 (0.56 %–0.98 %), Mg# values (52–76), Th/Ce (0.05–0.17) ratios and high but variable Ni contents (24 ppm – 178 ppm), which are similar to subducted slab-derived adakitic melts (Fig. 13) but distinct from the thickened or delaminated lower crust-derived adakitic rocks (Chung et al. Reference Chung, Liu, Ji, Chu, Lee, Wen, Lo, Lee, Qian and Zhang2003; Wang et al. Reference Wang, Xu, Jian, Bao, Zhao, Li, Xiong and Ma2006). (2) Their REE and trace-element patterns are similar to those of the adakitic-like Karamay – Baogutu saukitoids, which are widely considered to be derived from the reaction between subducting oceanic slab and the peridotite mantle (Fig. 10) (Yin et al. Reference Yin, Yuan, Sun, Long, Zhao, Wong, Geng and Cai2010, Reference Yin, Long, Yuan, Sun, Zhao and Geng2013); (3) However, mechanisms involving over-thickened and delaminated lower crust are not favoured by their high positive εNd values (+4.54 – +7.75). (4) Group 1 dykes have rather high Sr concentrations (491 ppm–789 ppm) with mean value of 660 ppm, which indicates that the mantle was probably metasomatized by the melts (Martin et al. Reference Martin, Smithies, Rapp, Moyen and Champion2005).
A plot of Th/Nd ratio vs. (143Nd/144Nd) i/εNd(t) (Johnson & Plank, Reference Johnson and Plank1999; Zhang et al. Reference Zhang, Liu, Xiao, Xu, Shi, Gong, Hu, Liu, Song, Xiao, Zhang, Li and Li2021b) can be used to model the contribution of sediment-derived melt and three-component mixing models (DePaolo et al. Reference DePaolo, Linn and Schubert1991) for the dykes are shown Fig. 15. This modelling shows that 1%–2% sediment/sediment partial melt and 0–5% trapped oceanic crust-derived melts mixed with a depleted mantle source could generate the compositions of the Group 1 dykes (Fig. 15).

Figure 15. Plot of Th/Nd versus 143Nd/144Nd(t) of the Carboniferous dykes in west Junggar. Subducted sediment from Plank et al. (Reference Plank, Kelley, Murray and Stern2007), Plank and Langmuir (Reference Plank and Langmuir1998), Nowell et al. (Reference Nowell, Kempton, Noble, Fitton, Saunders, Mahoney and Taylor1998) and Vervoort et al. (Reference Vervoort, Plank and Prytulak2011). DMM trace-element concentrations are from Su and Langmuir (Reference Su and Langmuir2003) and Workman and Hart (Reference Workman and Hart2005). Sediment/melt and sediment/fluid partition coefficients from Johnson and Plank (Reference Johnson and Plank1999). The trapped oceanic crust was represented by klm28 (metagabbro) from Zhu et al. (Reference Zhu, Chen and Qiu2015). The Sanukitoid data of the Karamay-Baogutu area are from Tang et al. (Reference Tang, Wang, Wyman, Li, Xu and Zhao2012a), Ma et al. (Reference Ma, Xiao, Windley, Zhao, Han, Zhang, Luo and Li2012) and Yin et al. (Reference Yin, Yuan, Sun, Long, Zhao, Wong, Geng and Cai2010, Reference Yin, Long, Yuan, Sun, Zhao and Geng2013).
The presence of residual accessory phases profoundly controls the trace-element composition of the adakitic melts. Therefore several characteristic trace-element concentrations and ratios are commonly regarded as proxies for residual accessory phases in magma sources and so can be used to evaluate the pressure and temperature conditions of melting. The Sr/Y and (La/Yb) N ratios of intermediate-felsic magmas are essentially controlled by the mineral phases (e.g., garnet, rutile, amphibole and plagioclase) in the residue or by fractionation crystallisation of parent magma. Group 1 dykes have relatively steep HREE patterns ((Gd/Yb) N = 1.24–2.26 mean value of 1.66) with high Sr/Y (31.68–82.85, mean value of 56.26) ratios, which are similar to the Karamay-Baogutu sanukitoids ((Gd/Yb) N = 1.11–2.56; Sr/Y = 26.18–129.53 mean value of 52) (Table S4), which were derived from a source containing some residual garnet and rutile (Yin et al. Reference Yin, Yuan, Sun, Long, Zhao, Wong, Geng and Cai2010, Reference Yin, Long, Yuan, Sun, Zhao and Geng2013; Ma et al. Reference Ma, Xiao, Windley, Zhao, Han, Zhang, Luo and Li2012; Tang et al. Reference Tang, Wang, Wyman, Li, Xu and Zhao2012a). However, Group 1 dykes show Nb-Ta negative anomalies but without distinct Ti depletion on a primitive mantle-normalised multi-element plot (Fig. 10b, d and h), suggesting that rutile is absent in the magma source. Ratios such as K/Yb and Dy/Yb can be used to distinguish between melting in the spinel and garnet stability field of phlogopite- and/or amphibole-bearing lherzolite (Duggen et al. Reference Duggen, Hoernle, Van den Bogaard and Garbe-Schonberg2005) because melting in the spinel and garnet stability field would produce melts with low Dy/Yb ratios (<1.5) and high Dy/Yb ratios (>2.5), respectively. Group 1 samples have Dy/Yb ratios of 1.60–2.24, indicating that they were probably derived from melting of a source in the spinel-garnet transition zone. As plagioclase is not a major fractionation phase and garnet is a residual in the source with fractionation of orthopyroxene and olive (Fig. 12d), Group 1 dykes have relatively high Sr/Y ratios. However, compared with typical adakitic rocks, the Group 1 dykes have low (La/Yb) N ratios, which was most likely caused by partial melting of peridotite mantle metasomatized by oceanic slab-derived adakitic melts (Ma et al. Reference Ma, Xiao, Windley, Zhao, Han, Zhang, Luo and Li2012; Yin et al. Reference Yin, Long, Yuan, Sun, Zhao and Geng2013). This indicates that the melt-mantle interaction may have diluted their adakitic features (Fan et al. Reference Fan, Wang, Li, Wei, Wyman, Zhao, Liu, Ma, Zhang and Wang2020). Based on a minimum pressure (1.5GPa) for rutile appearance, the depth for Group 1 sample production via melting of subducted oceanic crust cannot exceed 50 km (Xiong et al. Reference Xiong, Cai, Niu, Chen, Wang, Zhao and Wu2005; Xiong, Reference Xiong2006). Using MORB as a source rock, phase-equilibrium modelling indicates that adakites are generated under P-T conditions of 1.0–1.6 GPa and 800–1000 °C (Wang et al, Reference Wang, Cascio, Liang and Xu2020). Thus, it is clear that Group 1 dykes were formed at relatively high-temperatures (800°C–1050°C) and relatively high-pressure conditions (<1.5 Gpa) (Defant & Drummond, Reference Defant and Drummond1990; Xiong, Reference Xiong2006; Yin et al. Reference Yin, Long, Yuan, Sun, Zhao and Geng2013).
5.b.3 Petrogenesis of Group 2 dykes
The Group 2 dykes have similar characteristics of high Mg# values, Ni and Cr concentrations, relatively low (87Sr/86Sr) i (0.7041–0.7044) and high εNd(t) values (+4.4–+5.7) to the Group 1 dykes, indicating that they are derived from depleted mantle (Rapp & Watson, Reference Rapp and Watson1995). They have high Th/La and low Nb/Th ratios, and plot in the forearc basalts area, suggesting an oceanic slab-related origin (Fig. 14e). Their high Th/Yb ratios relative to MORB (Fig. 14f), positive high εNd(t) values and young Nd model ages (T DM ) indicate a juvenile depleted mantle source. Three main models for the formation of sanukitic HMAs in the Setouchi volcanic belt have been suggested: (1) Peridotite mantle assimilated by the intermediate-felsic magma (i.e., TTG-like melts) at crustal depths in intra-continental setting (Qian & Hermann, Reference Qian and Hermann2010). (2) Remelting of mantle-wedge peridotite modified by interaction with aqueous fluids derived from slab dehydration (Tatsumi & Hanyu, Reference Tatsumi and Hanyu2003) or (3) subducting sediments (Shimoda et al. Reference Shimoda, Tatsumi, Nohda, Ishizaka and Jahn1998; Tatsumi & Hanyu, Reference Tatsumi and Hanyu2003).
In relation to model (1), Qian and Hermann (Reference Qian and Hermann2010) proposed that intermediate-felsic magmas were formed in an extensional setting by partial melting of lower crust followed by reaction of this melt with peridotite at middle or upper crustal levels. Petrographic observations and geochemical compositions of Group 2 samples (Figs. 4 and 9) differ from those HMAs generated by model 1, which usually comprise a certain amount of olivine xenocrysts or dunite xenoliths and display adakitic-like compositions with high Sr, Ba but strong Nb-Ta depletions (Qian & Hermann, Reference Qian and Hermann2010). Furthermore, there is no evidence in the area for the emplacement of peridotite at middle or upper crustal levels, and thus this model does not represent a feasible mechanism for the generation of the West Junggar dykes.
We propose that Group 2 dykes were derived from remelting of mantle-wedge peridotite modified by interaction with subducting sediments rather than aqueous fluids based on following evidence: (1) They possess high MgO, Mg#, Ni and Cr contents than typical island-arc andesites (Tatsumi, Reference Tatsumi2006). (2) On the MgO vs. SiO2 diagram (Fig. 8a), Group 2 samples show similarities to sanukitic HMAs in the Setouchi volcanic belt (Tatsumi, Reference Tatsumi2001; Yin et al. 2015), not only reflecting the differences in initial compositions compared with Group 1 samples but also suggesting a similar formation mechanism to Setouchi sanukitoids, which were generated by interaction between the peridotite mantle and sediment-derived melt in a young, or hot, subduction zone setting (Furukawa & Tatsumi, Reference Furukawa and Tatsumi1999). (3) The concentration of Th in marine sediments is more than two orders of magnitude higher than that of the mantle, and therefore the addition of sediment to the mantle will lead to increasing Th/La, Th/Sm and Th/Y ratios and decreasing (Nb/Th) ratios but will have little effect on Ta/Yb ratios (Plank & Langmuir, Reference Plank and Langmuir1998; Liu et al. Reference Liu, Xu, Castillo, Xiao, Shi, Feng and Guo2014). Group 2 have high Th/Yb and low Ba/La ratios, suggesting that they were generated by partial melting of the peridotite mantle metasomatized by sediment-derived melts, which is supported by the fact that they plot along a mixing line between the mantle and sediments on a Th/La vs. Sm/La, Th/La vs. Th and Th/Yb vs. Th/Sm and (Th/La)N-MORB vs. (Nb/Th)N-MORB diagrams, further suggesting significant interaction between subducting sediments and peridotite mantle (Fig. 14b, c and d). This depleted source had possibly undergone metasomatic enrichment by sediment-derived melt, resulting in an enriched-LILE and depleted-HFSE signature (Fig. 10e, f). (4) Fig. 15 indicates that addition of 2%–3.5% sediment/sediment partial melt to depleted mantle can model the composition of sanukitic Group 2 dykes in central West Junggar (Johnson & Plank, Reference Johnson and Plank1999; Zhang et al. Reference Zhang, Liu, Xiao, Xu, Shi, Gong, Hu, Liu, Song, Xiao, Zhang, Li and Li2021b).
Group 2 samples have Dy/Yb ratios of 1.61–2.08, indicating that like Group 1 dykes they were probably derived from melting of a source in the spinel-garnet transition zone. In Fig. 9d, Group 2 samples show no obvious Ti depletion indicating that rutile is not the residual phase. They have relatively flat HREE patterns ((Gd/Yb) N = 1.19 – 1.50 with mean value of 1.47 (Fig. 10e), low Sr/Y ratios of 18.67 – 33.9 (mean value of 24.07) and relatively low (La/Yb) N ratios, suggesting that there was little residual garnet in their source region. Moderate Y/Yb and (Ho/Yb) N ratios indicate that the residual phase in the source region of Group 2 samples is mainly amphibole rather than garnet (Wu et al. Reference Wu, Ge, Sun, Xiao, Deng and Ma2002). They display noticeable positive Sr anomalies and negligible negative Eu anomalies demonstrating that plagioclase was a residue in the source region (Fig. 10e, f). The phase-equilibrium between the melts and the plagioclase and amphibole during the partial melting of the basalts constrained by experimental petrology indicates that they were formed at pressures lower than 0.8 GPa (Ge et al. Reference Ge, Li, Chen and Li2002; Wu et al. Reference Wu, Ge, Sun, Xiao, Deng and Ma2002). The Bieluagaxi dykes (Group 2) give Ti-in-zircon crystallisation temperatures between 783°C and 1013°C (Ferry & Watson, Reference Ferry and Watson2007), which is similar to the crystallisation temperatures of the Group 1 samples.
Thus, Group 2 dykes were most likely derived from partial melting of peridotite mantle metasomatized by sediment-derived melts at a shallow depth but relatively high temperatures.
5.c Tectonic setting
Widespread Cambrian-Ordovician ophiolites and overlying Ordovician-Silurian volcanic-sedimentary rocks in Northern Xinjiang indicate that an early Palaeozoic Junggar Ocean existed in this region (Xu et al. Reference Xu, Ji, Zhao, Gong, Zhou, He, Zhong, Wang and Griffiths2013). The age span of ophiolites or ophiolitic mélanges in West Junggar ranges from 572±25 Ma to 332±14 Ma (Zhang et al. Reference Zhang, Wang, Polat, Zhu, Shen, Chen, Chen, Guo, Wu and Liu2018 and references therein). Daerbute and Karamay ophiolitic mélanges in central West Junggar (Fig. 2) are thought to be formed in a subduction-related setting from 426 Ma to 375 Ma (Chen & Zhu, Reference Chen and Zhu2011; Yang et al. Reference Yang, Li, Santosh, Gu, Yang, Zhang, Wang, Zhong and Tong2012b) and from 414 Ma to 363 Ma, respectively (Xu et al. Reference Xu, He, Li, Ding, Liu and Mei2006; Yang et al. Reference Yang, Li, Santosh, Yang, Zhang and Tong2013). In addition to the ophiolite complexes and mélanges, this subduction in the Early Carboniferous is evidenced by granitoids (e.g., Han et al. Reference Han, Ji, Song, Chen and Zhang2006), dyke swarms (e.g., Tang et al. Reference Tang, Wang, Wyman, Li, Xu and Zhao2012a) and volcanic rocks (e.g., Geng et al. Reference Geng, Sun, Yuan, Zhao and Xiao2011), which have island arc signatures (Feng et al. Reference Feng, Coleman, Tilton and Xiao1989; Xiao et al. Reference Xiao, Han, Yuan, Sun and Lin2008).
Various tectonic settings of West Junggar in Late Carboniferous have been proposed from post-collisional (Chen & Arakawa, Reference Chen and Arakawa2005; Han et al. Reference Han, Ji, Song, Chen and Zhang2006; Chen et al. Reference Chen, Han, Ji, Zhang, Xu, He and Wang2010; Xu et al. Reference Xu, Ji, Zhao, Gong, Zhou, He, Zhong, Wang and Griffiths2013; Gao et al. Reference Gao, Xiao, Pirajno, Wang, He, Yang and Yan2014; Zheng et al. Reference Zheng, Han, Wang, Liu and Feng2020), to subduction-accretionary (Windley et al. Reference Windley, Alexeiev, Xiao, Kroner and Badarch2007; Xiao et al. Reference Xiao, Han, Yuan, Sun and Lin2008) to ridge subduction settings (Geng et al. Reference Geng, Sun, Yuan, Xiao, Xian, Zhao, Zhang, Wong and Wu2009; Tang et al. Reference Tang, Wang, Wyman, Li, Zhao, Jia and Jiang2010, Reference Tang, Wang, Wyman, Li, Xu and Zhao2012a; Ma et al. Reference Ma, Xiao, Windley, Zhao, Han, Zhang, Luo and Li2012; Yin et al. Reference Yin, Yuan, Sun, Long, Zhao, Wong, Geng and Cai2010, Reference Yin, Long, Yuan, Sun, Zhao and Geng2013, Reference Yin, Chen, Xiao, Yuan, Sun, Tang, Yu, Long, Cai, Geng, Zhang and Liu2015a). Arc magmatism ceased at the beginning of Late Carboniferous and the wide spatial distribution of granitoids formed in West Junggar during Late Carboniferous – Early Permian does not fit with the narrow and linear distribution pattern of magmatism that would be expected if slab break-off had occurred (Whalen et al. Reference Whalen, McNicoll, van Staal, Lissenberg, Longstaffe, Jenner and van Breeman2006; Li et al. Reference Li, He and Fan2015). Both group dykes are characterised by enrichment in LILEs and depletion in HFSEs and significant negative Nb anomaly showed by the two groups of dykes (Fig. 9) reveals that they may have been formed in a subduction-related setting (Rudnick & Gao, Reference Rudnick and Gao2003) (Fig. 10). However, no record of oceanic crust and accretion-collision processes or (ultra-)high-pressure metamorphism younger than 300 Ma have been reported, indicating that the subduction-related setting is not a good choice (Liu et al. Reference Liu, Han, Xu, Ren and Chen2019). Thus, based on following pieces of evidence, we propose a post-collisional setting in West Junggar since Late Carboniferous:
(1) Choulet et al. (Reference Choulet, Faure, Cluzel, Chen, Lin and Wang2012) argued that accretion and subduction in the West Junggar must have ceased during the Late Carboniferous with transition from an accretionary to a post-accretionary setting between 305 Ma – 300 Ma, which is consistent with the lack of evidence for Permian subduction in the sedimentary or tectonic records (Buckman & Aitchison, Reference Buckman and Aitchison2004; Choulet et al. Reference Choulet, Faure, Cluzel, Chen, Lin and Wang2012). (2) Early Carboniferous magmatism has been ascribed to this subduction-accretion event, which then developed into an intra-continental orogenic event both in the northern and southern West Junggar region during the late Early Carboniferous to the early Late Carboniferous (Duan et al. Reference Duan, Li, Zhi, Yang and Gao2019). (3) Late Carboniferous collisional events between different tectonic blocks (e.g., Yili-Chinese central Tianshan block and Junggar terrane along the Chinese north Tianshan suture zone) (Zhang et al. Reference Zhang, Zhao, Sun, Eizenh€ofer, Han, Hou, Liu, Wang, Liu and Xu2016; Han & Zhao, Reference Han and Zhao2018) in northern Xinjiang, led to the closure of the West Junggar remnant ocean basin and other sub-oceans of the Palaeo-Asian Ocean (Han & Zhao, Reference Han and Zhao2018). The final closure of the Junggar Ocean likely occurred at the end of the Late Carboniferous in response to regional amalgamation events in the southwestern CAOB, representing the final assembly of the Kazakhstan Orocline (Zhang et al. Reference Zhang, Wang, Shen, Polat and Zhu2021a). (4) Undeformed mafic – intermediate dykes dissect the deformation structures including folds and faults, which were correlated with subduction-accretionary process in Early Carboniferous, present that the cessation of subduction-accretionary process when the abundances of dykes were emplaced (Zhan et al. Reference Zhan, Hou, Hari and Shu2015). Because these dykes could be deemed as ‘stitching rocks’, they were formed after the deformation caused by subduction-accretionary process in Early Carboniferous. (5) Stress measurements on the studied dykes (172 in total) were obtained during field work and calculated by method from Delvaux and Spencer (Reference Delvaux, Sperner and Nieuwland2003). All dykes were formed in an extensional stress regime including pure, oblique and strike-slip extensional regimes (Table S8). This is consistent with the conclusion that the mafic – intermediate dykes in West Junggar formed in within-plate extensional setting, coeval with the widespread lithospheric extensional events in the Northern Xinjiang (Coleman, Reference Coleman1989; Jahn et al. Reference Jahn, Wu and Chen2000; Han et al. Reference Han, Ji, Song, Chen and Zhang2006; Zhan et al. Reference Zhan, Hou, Hari and Shu2015). (6) Widespread deposition of Permian continental molasse deposits followed the formation of post-collisional magmatic rocks (Zong et al. Reference Zong, Wang, Jiang and Gong2016). The Gzhelian Molaoba Formation consisting of non-marine fluvial sediments represents the central West Junggar back-arc basin that finally closed by the Late Carboniferous (Liu et al. Reference Liu, Han, Chen, Ren, Zheng, Wang and Feng2017). (7) Abundances of Late Carboniferous – Early Permian undeformed A-type granitic intrusions in West Junggar (emplacement ages between 305 Ma and 280 Ma) have highly-depleted Hf-Nd isotopic affinities. They have been proposed to be derived from depleted mantle or juvenile basaltic lower crust that was generated in an extensional post-collisional setting (e.g., Xu et al. Reference Xu, Ji, Zhao, Gong, Zhou, He, Zhong, Wang and Griffiths2013; Gao et al. Reference Gao, Xiao, Pirajno, Wang, He, Yang and Yan2014; Zheng et al. Reference Zheng, Han, Wang, Liu and Feng2020). Minor contemporaneous I-type granites were derived from the differentiated products of the basaltic melts or a mixture between basaltic melts and juvenile lower crust (Chen et al. Reference Chen, Han, Ji, Zhang, Xu, He and Wang2010). The Late Carboniferous – Permian granitoids in West Junggar (Table S7) are plotted in the VAG and Post-COLG settings, which show transitional affinities (Fig. 16). They were probably formed in a transitional background from subductional to post-collisional settings. Additionally, the dykes were generated a little later than the host granitoids, indicating that they were probably formed in a post-collisional setting.

Figure 16. Tectonic discrimination diagrams of (a) Nb vs. Yb (Pearce et al. Reference Pearce, Harris and Tindle1984) and (b) Rb vs. Y+Nb (Whalen et al. Reference Whalen, Currie and Chappell1987). ORG, ocean-ridge granites; post-COLG, post-collision granites; syn-COLG, syn-collision granites; VAG, volcanic arc granites; WPG, within-plate granites. All the data of Late Carboniferous to Early Permian granitoids are listed in Table S7 from Gao et al. (Reference Gao, Xiao, Pirajno, Wang, He, Yang and Yan2014) and references therein.
5.d Magmatic flow and emplacement of dykes
5.d.1 Magmatic flow of dykes
Syn-tectonic magmatic flow is defined as deformation by melt displacement with consequent rigid-body crystal rotation, but without sufficient interference between crystals to cause plastic deformation (Paterson et al. Reference Paterson, Vernon and Tobisch1989). Although flow structures in dykes and plutons are generally seldom observed at outcrop or under the microscope, they include alignment of bubbles or phenocrysts in dykes, orientation and strain of enclaves, hot striations on the contact, magmatic foliation or lineation in plutons, rarely ropy flow structure and other structures (Baer, 1987; Tian & Shan, Reference Tian and Shan2011; Zhou & Wang, Reference Zhou and Wang2012). All above are useful to determine the flow direction of magma, thus helping understand the processes responsible for magma mixing or segregation during flow, nucleation and propagation of dykes.
Flow structures observed in Bieluagaxi dykes rather than other dykes in West Junggar are mainly magmatic bands and folds. These structures were produced in the magma body as a result of flow-induced shearing within the dykes. In outcrop, the magmatic bands appear dark grey or light white in colour, with a variable amount of euhedral or subhedral hornblende and plagioclase and/or quartz crystals of several millimetres in diameter. They are generally parallel or subparallel to the boundaries of dykes (Fig. 3i and j) but are not always parallel to each other, and they sometimes may make up rootless isocline folds (Fig. 3j). The contact between the bands and their ambient rocks is straight, slightly undulating or occasionally zigzag (Fig. 3i and j). As distinguishing the folding mechanisms is difficult or even impossible due to the insufficient observations at most outcrops, we just describe two interesting types: injection folds and disharmonic folds. The former appears at the blunt end of the dyke (Fig. 3j). Generally, they are isoclinal with the axial plane oriented parallel or subparallel to the sides of dykes. However, in the Fig. 3j, this injection fold occurs in local part of the dyke. The disharmonic fold has a variable size and thickness with respect to the limbs (Fig. 3h). Neither foliation nor cleavage is visible in the cores of this tight fold, suggesting the disturbance of flow-induced shearing by continuous laminar flow after magmatic bands formed in dykes.
In this study, the magmatic banding is generally considered a product of magma mingling due to the fact that most of the bands are parallel or subparallel to the contact. The megacryst K-feldspar has poikilotopic texture that euhedral amphiboles and acicular apatites embedded in the plagioclase (Fig. 4g), which is also supported by the plagioclase xenocrysts (Fig. 3g), dioritic xenolith (Fig. 3h) and round-to-elongated-shaped granitic enclaves (Fig. 3k) in dykes, indicating that the magma of the dioritic dyke was initially quenched into the unconsolidated host granitic magma and mingled with it to a certain extent.
5.d.2 Emplacement of dykes
The strike and dip orientation analysis of the dyke swarm confirms the prevalence of sub-vertical and sub-horizontal WNW-trending dykes (Late 1 stage) with minor NE- (Late 2 stage) and ENE-trending (Early stage) dykes throughout the entire study area (Fig. 2, S2; Table S1). The WNW- and NE-trending sub-vertical and sub-horizontal dyke orientation and ENE-trending (Early stage) sub-vertical dyke orientation appear to coincide with one of the two main fracture sets (NE-SW and WNW-ENE) in the host rocks (Fig. 3a, c, S3c, e, i and j) in Karamay, Xiaerpu and Bieluagaxi areas, and with localised NE-ENE-trending fractures or faults in Liushugou area (Fig. 3e, S3k), respectively, a fact that is compatible with the possibilities that most dykes could have exploited pre-existing joints/faults or that dykes and dyke/parallel joints/faults are contemporaneous.
Variations in strike and/or dip have also been observed along individual dykes, revealing a continuous zigzagging stepped geometry (Fig. 3a, S3c, e, g–i) and are probably equally related to the exploitation of joints (Martinez-Poza & Druguet, Reference Martinez-Poza and Druguet2016). The joints/faults within the host granitic rocks and surrounding Upper Carboniferous strata were generated in an extensional regime in Late Carboniferous. The joints/faults show tensile characteristics in the field (Fig. S3b, f, g, h and j). Thus, the tensile joints/fractures of the host granitic rocks and surrounding strata were formed in that extensional setting in Late Carboniferous, immediately or in a very short time after being filled by magma propagation. As discussed in section 5.d.1, the magmas of dioritic dyke were initially quenched into the unconsolidated host granitic magma, indicating that the emplacement interval between the dykes and host granites may have been very short, which is also indicated by their similar ages. In other words, these pre-existing joints/fractures were formed after the emplacement of granitoids in a tensional regime in Late Carboniferous, and the time interval between these pre-existing joints/fractures and dykes may be probably only a few million years. Thus, these joints/fractures could be deemed as the ‘contemporaneous’ products with dykes in central West Junggar. A plausible model is that ‘contemporaneous’ joints in the granitoids were exploited during dyke emplacement and subsequently reactivated during cooling and post-cooling events, implying active tectonics during and after the intrusion of the dyke swarm (Martinez-Poza & Druguet, Reference Martinez-Poza and Druguet2016).
The dykes are parallel and they were formed in response to the regional tensile stress field (Hou, Reference Hou2012). Our paleostress analysis gives an estimation of the orientation and relative magnitude of the tectonic principal stress axes operating at the time of intrusion (Fig. S2). To Karamay and Bieluagaxi dykes, the σ1 was sub-vertical, and σ2 and σ3 sub-horizontal, whereas the σ2 of the dykes in Xiaerpu and Liushugou areas were sub-vertical with σ1 and σ3 sub-horizontal (Table S8; Fig. S2). There is a good correspondence between these paleostress values and field structures observed between dyke patterns in horizontal and vertical sections. Emplacement of the dykes took place in the form of extension fractures during a regional extensional tectonic regime with strike-slip, as inferred from the sub-vertical and sub-horizontal principal stress axes (σ1). Dykes preferentially opened perpendicular to their walls. The estimated average extension (T) accommodated by dyke intrusion is considerable as 2.7% (Li et al. Reference Li, Han, Li, Liu and Du2005). Numerous dyke swarms, found in West Junggar, show similar geochemical and isotopic affinities of a post-collisional setting in Late Carboniferous. It is therefore likely that there is a magma chamber of relevant components in the deep part of the dyke.
Thus, the dyke intrusion extensional seems to have been favoured by the presence of ‘contemporaneous’ joints or fractures/faults in the host granitic rocks or strata. The final pattern of dykes likely resulted from the interaction between these fractures and the external tectonic stress tensor active during dyke emplacement.
5.e Tectonic implications
In West Junggar, a Late Carboniferous magmatic association of various volcanic and intrusive rocks is extensively developed and is mostly derived from a mantle magmatic source (Chen & Jahn, Reference Chen and Jahn2004; Geng et al. Reference Geng, Sun, Yuan, Xiao, Xian, Zhao, Zhang, Wong and Wu2009; Chen et al. Reference Chen, Han, Ji, Zhang, Xu, He and Wang2010; Yin et al. Reference Yin, Yuan, Sun, Long, Zhao, Wong, Geng and Cai2010; Zheng et al. Reference Zheng, Han, Wang, Liu and Feng2020). Late Carboniferous (306 Ma – 315 Ma) N-MORB to OIB-like tholeiitic and alkaline basalts in Yeyagou, Maliya and Hatu were discovered and interpreted as derived from various enriched mantle sources (Zhang et al, Reference Zhang, Xiao, Han, Ao, Yuan, Sun, Geng, Zhao, Guo and Ma2011a, Reference Zhang, Xiao, Han, Mao, Ao, Guo and Ma2011b) or from a mixed mantle source consisting of subducted depleted oceanic lithosphere and enriched upwelling asthenospheric mantle associated with ridge-trench interaction (Tang et al. Reference Tang, Wyman, Wang, Li, Li, Zhao and Sun2012c). However, recent work has shown that the Junggar basin is composed of (1) an assemblage of Late Palaeozoic arc fragments, accretionary complexes and trapped oceanic crust (Li et al. Reference Li, He and Fan2015), or (2) oceanic crust (Xu et al. Reference Xu, Ji, Zhao, Gong, Zhou, He, Zhong, Wang and Griffiths2013) consistent with the idea that the basement of the West Junggar region is mainly composed of slices of oceanic crust (Zheng et al. Reference Zheng, Sun, Zhao, Robinson and Wang2007; Zhang et al. Reference Zhang, Wang, Polat, Zhu, Shen, Chen, Chen, Guo, Wu and Liu2018) with minor Precambrian basement (Hu et al. Reference Hu, Jahn, Zhang, Chen and Zhang2000). Existing geophysical data show that the Junggar Basin is characterised by high velocity, density and magnetisation (Zhao et al. Reference Zhao, Huang, Ma, Shao, Cheng, Wang and Xu2008). A preserved Palaeo-oceanic lithosphere, which is becoming a piece of Eurasian continent beneath the Junggar region, has been presented by seismic evidence (Wu et al. Reference Wu, Yang, Xu, Afonso and Zhang2023), which suggests that the basement of the Junggar Basin is oceanic crust.
Post-collisional magmatism was triggered by asthenosphere upwelling resulting in the widespread formation of Late Carboniferous and Permian granitoids and dykes in West Junggar and adjacent regions, e.g., Altay, Tianshan and North Tianshan (Han et al. Reference Han, Ji, Song, Chen and Zhang2006; Zheng et al. Reference Zheng, Han, Wang, Liu and Feng2020). This interpretation is supported by Sr-Nd isotopic signatures of the magmatic rocks in West Junggar, which show widespread development of juvenile crust with minimal contribution of Precambrian rocks that preclude the occurrence of TTG (Zheng et al. Reference Zheng, Han, Wang, Liu and Feng2020). The generation of the HMAs needs high-temperature conditions to trigger partial melting of the subducted slab and sediments (Tatsumi, Reference Tatsumi2008). Considering the occurrence of high Sr/Y type HMAs and sanukitic dykes with those tholeiitic and alkaline basalts in West Junggar, they are not only the indicator of an extensional setting but also a high-temperature geothermal gradient. Unlike Archean HMAs, commonly considered to be produced at a very high geothermal gradient (Martin et al. Reference Martin, Smithies, Rapp, Moyen and Champion2005), the Phanerozoic HMAs such as the Late Carboniferous sanukitic dykes in West Junggar require special dynamic processes to elevate the geothermal gradient of the post-Archean upper mantle (Shimoda et al. Reference Shimoda, Tatsumi, Nohda, Ishizaka and Jahn1998).
There are two possible tectonic scenarios for raising the geothermal gradient, i.e., lithospheric delamination or subducted slab break-off (Liu et al. Reference Liu, Han, Chen, Ren, Zheng, Wang and Feng2017). Both processes may result in asthenosphere upwelling and coeval basaltic underplating, leading to the partial melting of oceanic crust. Delamination may produce non-linear diffuse distribution of magmatism in a large area, whereas the magmatism caused by slab break-off will be confined to a narrow linear zone with significant crustal uplift (Whalen et al. Reference Whalen, McNicoll, van Staal, Lissenberg, Longstaffe, Jenner and van Breeman2006; Liu et al. Reference Liu, Han, Chen, Ren, Zheng, Wang and Feng2017). The spatial-temporal pattern of Carboniferous magmatism in West Junggar shows a tendency to southward-younging in West Junggar (Zhang et al. Reference Zhang, Wang, Shen, Polat and Zhu2021a), which makes slab break-off model more feasible. This suggestion is also consistent with the uplift of the Junggar terrane remnant oceanic crust during its final development between 335 Ma and 333 Ma (Zhu et al. Reference Zhu, Chen and Qiu2015). The Late Carboniferous adakitic rocks and HMA-like rocks from the Karamay and Baogutu region could also be produced by partial melting of the broken-off oceanic crust during the initial phase of orogenic collapse (Ren, Reference Ren2013).
In the Early Carboniferous, the back-arc basin, which resulted from northwest-ward intra-oceanic subduction of the Junggar Ocean in the Zhongguai area between the Junggar complex basement and the Yili block (Zhang et al. Reference Zhang, Wang, Polat, Zhu, Shen, Chen, Chen, Guo, Wu and Liu2018), started to subduct with generation of arc-related I-type granites. Numerous volcano-clastic and tuffaceous materials from Carboniferous arc-related rocks were deposited quickly in this back-arc basin (Zhang et al. Reference Zhang, Wang, Shen, Polat and Zhu2021a). During subduction, the oceanic slab underwent break-off resulting in asthenosphere upwelling and the tectonic regime changed from compression to an extensional post-collisional setting in the early Late Carboniferous. The trapped oceanic-crust-like basement of West Junggar was heated by the asthenospheric mantle and began to melt. Thus, Bieluagaxi (324 Ma) dykes were derived from the reaction between the peridotite mantle and the sediment-derived melts at a shallow depth but low temperature.
Magmatic activity mostly occurred between ca. 332 Ma and 287 Ma based on the systematic combining and summarising of available zircon U-Pb chronological data of granitoids in West Junggar (Duan et al. Reference Duan, Li, Zhi, Yang and Gao2019). During this period, the magmatism was successive and showed two important phases of magmatic activities with peak ages of ca. 314 Ma and ca. 302 Ma. During this period, many magmatic rocks including A-type granites, adakitic-like and sanukitic-like rocks with minor tholeiitic, alkaline and Nb-enriched-like rocks were produced during the mantle-crust interaction process in the post-collisional regime. In Late Carboniferous, the slab-derived melt with adakitic affinity interacted with peridotite mantle and sediment-derived melts resulting in the formation of Group 1 dykes along the joints and fractures to transport and propagate. The Carboniferous strata were then folded during the tectonic extrusion of the Daerbute and Karamay ophiolitic mélanges because the central West Junggar terrane, which was situated in the core area of the Kazakhstan Orocline, underwent tectonic uplift and extensive deformation (Zhang et al. Reference Zhang, Wang, Polat, Zhu, Shen, Chen, Chen, Guo, Wu and Liu2018).
5.f Phanerozoic Continental crustal growth
The Canadian – Alaskan Cordillera of North America and the CAOB are two large Phanerozoic orogenic belts that contain significant accounts of juvenile materials (Patchett & Samson, Reference Patchett and Samson2003). The CAOB was built by the subduction and closure of the Palaeo-Asian Ocean and is surrounded by the Siberian craton to the north, and the Tarim and North China cratons to the south and the European craton to the west (Zheng et al. Reference Zheng, Han, Wang, Liu and Feng2020). The West Junggar orogenic belt is correlated with arc-related magmatism and accretion of oceanic rocks from latest Ediacaran to Early Carboniferous and was associated with asthenosphere upwelling-related magmatism in post-collisional setting during Late Carboniferous to Permian (Gao et al. Reference Gao, Xiao, Pirajno, Wang, He, Yang and Yan2014; Zheng et al. Reference Zheng, Han, Wang, Liu and Feng2020).
In West Junggar, widespread Late Carboniferous – Early Permian magmatism is mostly characterised by primitive εHf(t) and εNd(t) values and Palaeozoic model ages, which indicate massive continental crust growth by recycling of oceanic crust or direct differentiation of basaltic underplated material that originated from melting of a metasomatized lithospheric mantle (Jahn et al. Reference Jahn, Wu and Chen2000; Han et al. Reference Han, Ji, Song, Chen and Zhang2006; Chen et al. Reference Chen, Han, Zhang, Xu, Liu, Qu, Li, Yang and Yang2015). It has been estimated that nearly 50% of the continental crust in the CAOB is juvenile and the granitoids (Table S7) with high positive εNd(t) values contain more than 50% mantle-derived or juvenile materials (Sengör et al. 1993; Jahn et al. Reference Jahn, Wu and Chen2000). The estimate of the proportion of depleted mantle in the mixture can be semi-quantified by using a simple two-end member mixing model (DePaolo et al. Reference DePaolo, Linn and Schubert1991). The majority of granitoids, dykes and enclaves in West Junggar plot between the crust and depleted mantle, and rocks with similar ages show approximately vertical trends, suggesting variable mixing of the crust and the depleted mantle (Fig. 11a and b) with large proportions of mantle components (Fig. 11c and d). The mantle components in Late Carboniferous igneous rocks including granitoids, dykes and enclaves in central West Junggar vary from 41.2% to 99.7% with average value of 84.6% (Fig. 9c and d). These post-collisional igneous rocks including studied samples with Sr-Nd isotopes characterised by young T DM and high positive εNd(t) values suggest considerable continental crustal growth in a post-collisional setting during Late Carboniferous to Early Permian.
6. Conclusions
-
(1) LA-ICP-MS zircon U-Pb dating results reveal that the dykes in central West Junggar were formed between 309.7±2.4 Ma and 324.3±3.6 Ma and show coeval relationships with their corresponding host rocks.
-
(2) The dykes are divided into two groups based on Sr/Y ratios: Group 1 including Karamay, Xiaerpu and Liushugou dykes with high Sr/Y ratios of 41–83, which are similar to the adakitic HMA; whereas Group 2 includes Bieluagaxi dykes with low Sr/Y ratios of 19–34, which resemble the sanukitic HMA.
-
(3) It is proposed that 1%–2% sediment/sediment partial melt and 0–5% trapped oceanic crust-derived melts mixed with a depleted mantle source could generate the compositions of the adakitic-like Group 1 dykes, and 2%–3.5% sediment/sediment partial melt interacting with the depleted mantle may form the sanukitic Group 2 dykes in West Junggar.
-
(4) Large-scale asthenosphere upwelling could explain much of the magmatism and substantial continental crustal growth of West Junggar in the Late Carboniferous-Early Permian.
Supplementary material
To view supplementary material for this article, please visit https://doi.org/10.1017/S0016756823000663
Acknowledgements
We are grateful for the constructive comments by two anonymous reviewers and editors. We thank Sun Yi for helping to improve our manuscript. The fieldwork was assisted by Du Pengchuan.
Funding statement
This study benefited from the National Natural Science Foundation of China (Grant No. 41403023); and Geochemical 3D Modelling and Forecasting of Deep Mining (Grant No. 2017YFC0601505).
Competing interests
None.