1. Introduction
The geological evolution of subduction zones is typically governed by recurrent tectonic and magmatic activities that result in the development of polyphase orogenic belts (Brown, Reference Brown, Cawood and Kröner2009). A detailed tectonothermal history and understanding of temporal relationships between various tectonic domains in an orogen not only provide insights into global tectonic processes but also help in reconstructing the palaeogeography of dispersed crustal fragments within supercontinents (Cawood & Buchan, Reference Cawood and Buchan2007). The orogenic systems are primarily classified into accretionary and collisional types (cf. Windley, Reference Windley1992). Accretionary orogens (Fig. 1a) are formed in response to the subduction of oceanic crust along continental margins (Windley, Reference Windley1992; Condie, Reference Condie, Hatcher, Carlson, McBride and Martínez Catalán2007), whereas collisional orogens (Fig. 1b) are developed during the orthogonal collision between two or more continental blocks producing a thickened and reworked crust (Windley, Reference Windley1992; Brown, Reference Brown, Cawood and Kröner2009). Collisional orogens juxtapose different cratons (Fig. 1b) and are always preceded by the development of accretionary orogens (Cawood & Buchan, Reference Cawood and Buchan2007). Based on kinematics and the resulting structural framework, accretionary orogens are further categorized into retreating and advancing types (Cawood & Buchan, Reference Cawood and Buchan2007). The growth of retreating orogens is characterized by faster rates of rollback of the subducting plate than the rates of advance of the overriding plate (Elsasser, Reference Elsasser1971, Schellart & Lister, Reference Schellart, Lister, Sussman and Weil2004), leading to the development of back-arc and intra-arc basins within the overriding plate (Dickinson, Reference Dickinson, Busby, Ingersoll and Burbank1995; Marsaglia, Reference Marsaglia, Busby, Ingersoll and Burbank1995, Smith & Landis, Reference Smith, Landis, Busby, Ingersoll and Burbank1995). On the other hand, advancing orogens develop in zones where the velocity of the overriding plate supersedes that of the subducting slab (Russo & Silver, Reference Russo and Silver1996; Silver et al. Reference Silver, Russo and Lithgow-Bertelloni1998; Schellart, Reference Schellart2008). Advancing orogens are characterized by crustal thickening, an extensive reworking of the pre-existing crust and the formation of fold and thrust belts (Cawood et al. Reference Cawood, Kröner, Collins, Kusky, Mooney, Windley, Cawood and Kröner2009). Despite the significant differences in operating mechanisms, the geodynamic framework and crustal growth along the accretionary margins are manifested through widespread magmatism and crustal thickening during multiple episodes of burial and exhumation (Lister et al. Reference Lister, Forster, Rawling, Miller, Holdsworth, Buick and Hand2001; Collins, Reference Collins2002; Beltrando et al. Reference Beltrando, Hermann, Lister and Compagnoni2007). Further, in some cases, slab break-off has been recognized as a dominant mechanism in the development of orogens during continental collision (Fig. 1c), which is commonly associated with changes in the rate of plate convergence, subduction polarity reversal and slab rollback (Garzanti et al. Reference Garzanti, Rade and Malusà2018 and references therein).

Fig. 1. Sketches illustrate types of orogens and mechanism of slab break-off: (a) accretionary orogen, (b) collisional orogen, and (c) slab break-off along a convergent margin. (d) Geological map of the CITZ (modified after Deshmukh et al. Reference Deshmukh, Prabhakar, Bhattacharya and Madhavan2017; Bhowmik, Reference Bhowmik2019) showing the spatial distribution of discrete lithological domains in the fold belt.
The Central Indian Tectonic Zone (CITZ; Fig. 1d) in the Indian peninsula, also known as the Satpura Orogen, marks the zone of convergence between the Bundelkhand–Aravalli cratonic mass (North Indian Craton, NIC) and the Dharwar–Bastar–Singhbhum cratonic mass (South Indian Craton, SIC) during Proterozoic time (Radhakrishna & Ramakrishnan, Reference Radhakrishna and Ramakrishnan1988; Radhakrishna, Reference Radhakrishna1989; Roy & Prasad, Reference Roy and Prasad2003). The diverse lithological domains in this orogen preserve crucial evidence of the subduction, collision and accretion between the NIC and SIC (Yedekar et al. Reference Yedekar, Jain, Nair and Dutta1990; Acharyya, Reference Acharyya2003; Roy & Prasad, Reference Roy and Prasad2003). The evolutionary history of the CITZ is thought to be a product of multiple orogenic events between Palaeoproterozoic and early Neoproterozoic times. The majority of the Palaeoproterozoic tectonic events are best preserved along the northern segment of the CITZ, while the late Mesoproterozoic – early Neoproterozoic events are mainly recognized within the southern parts of the CITZ (Deshmukh & Prabhakar, Reference Deshmukh and Prabhakar2019). However, the direction of subduction and the initial timing of accretion between the NIC and the SIC remain disputed (Bhowmik et al. Reference Bhowmik, Wilde, Bhandari, Pal and Pant2012 and references therein). Based on existing models, some researchers believe that the SIC subducted northward beneath the NIC (Roy & Prasad, Reference Roy and Prasad2003; Mall et al. Reference Mall, Reddy and Mooney2008; Mandal et al. Reference Mandal, Sen and Vijaya Rao2013; Chattopadhyay et al. Reference Chattopadhyay, Chatterjee, Das and Sarkar2017), while some have argued in favour of southward subduction of the NIC beneath the SIC (Yedekar et al. Reference Yedekar, Jain, Nair and Dutta1990; Acharyya, Reference Acharyya2003; Bora, Reference Bora2015; Bhowmik & Chakraborty, Reference Bhowmik and Chakraborty2017). In both cases, the final closure of the ocean basin and the cessation of tectonic activity between the NIC and SIC is postulated at c. 1.0–0.9 Ga (Bhowmik et al. Reference Bhowmik, Wilde, Bhandari, Pal and Pant2012 and references therein). These events have a significant bearing on the geological evolution and transition of the tectonic regime from the initial to the final stages of the CITZ development. Spatially and temporally, the prominent tectonic episodes of the CITZ broadly coincide with the assembly and break-up of the Columbia and Rodinia supercontinents (Fig. 2; Bhowmik, Reference Bhowmik2019 and references therein).

Fig. 2. Relative probability plots of monazite ages of Mahakoshal Belt (MB) pelites and associated litho-units (modified after Deshmukh et al. Reference Deshmukh, Prabhakar, Bhattacharya and Madhavan2017 and Deshmukh & Prabhakar, Reference Deshmukh and Prabhakar2019). The sketches in the lower panel demonstrate the time-constrained deformation textures and associated mineral assemblages in andalusite and garnet–staurolite schists, where S1, S2 and S3 are denoted by dashed-dotted, solid and dashed lines, respectively. All mineral abbreviations are after Whitney & Evans (Reference Whitney and Evans2010). The tectonothermal events identified from the MB largely coincide with the timing of formation and subsequent dispersal of Columbia and Rodinia supercontinent assemblies (top panel).
In this contribution, we integrate our recent tectonothermal findings from the Mahakoshal Belt (MB; Fig. 2) with the existing data available from the CITZ and present a model for basin formation, sedimentation, metamorphism and magmatism associated with Proterozoic accretion along the fold belt. In combination with arguments made by earlier researchers, our hypothesis highlights the significance of recent geochronological and geophysical studies while describing the important tectonic events in the CITZ. We propose that the subduction polarity reversal between the NIC and SIC is responsible for the crustal growth and present tectonic framework of the CITZ, which involves an initial (Palaeo- to Mesoproterozoic) southward subduction of the NIC followed by the flipping of subduction polarity between late Mesoproterozoic and early Neoproterozoic times.
2. Tectonic framework of the CITZ
The E–W-trending CITZ (Fig. 1d) is a collage of granulite facies para/ortho-gneisses and greenschist- to amphibolite-facies volcano-sedimentary supracrustal sequences with distinct geological histories (Bhowmik et al. Reference Bhowmik, Pal, Pant and Shome1999; Roy & Prasad, Reference Roy and Prasad2003; Roy et al. Reference Roy, Kagami, Yoshida, Roy, Bandyopadhyay, Chattopadhyay, Khan, Huin and Pal2006). Other lithological units associated with these belts include the Tirodi Biotite Gneisses (TBG), Chottanagpur Gneissic Complex and sporadic granitoid intrusions. The Son-Narmada North Fault and the Central Indian Shear (CIS) represent the northern and southern boundaries of the CITZ, respectively (Fig. 1d) (Radhakrishna & Ramakrishnan, Reference Radhakrishna and Ramakrishnan1988; Ramakrishnan & Vaidyanadhan, Reference Ramakrishnan and Vaidyanadhan2010).
2.a. Granulite belts
The CITZ is composed of three major granulite terranes: the Makrohar Granulite (MG) belt to the north, the Balaghat–Bhandara Granulite (BBG) belt to the south and the Ramakona–Katangi Granulite (RKG) belt in the central part (Fig. 1d). These granulite belts comprise a variety of lithodemic units that preserve protracted Proterozoic tectonothermal histories (Roy & Prasad, Reference Roy and Prasad2003; Roy et al. Reference Roy, Kagami, Yoshida, Roy, Bandyopadhyay, Chattopadhyay, Khan, Huin and Pal2006; Bhowmik et al. Reference Bhowmik, Wilde, Bhandari and Sarbadhikari2014) and are spatially associated with low- to medium-grade supracrustal rocks (Fig. 1d). According to Roy & Prasad (Reference Roy and Prasad2003), the MG belt comprises intercalations of mafic and felsic lithologies (mafic granulite, banded iron formation and calc-silicates), which are abundantly exposed around the Makrohar area. Owing to the absence of direct geochronological data, the age of granulite metamorphism in the MG belt has been inferred at 1.8–1.7 Ga (Fig. 3), using the results obtained from Rb–Sr dating of the host granitoid (Sarkar et al. Reference Sarkar, Sarkar, Paul and Mitra1990; Acharyya, Reference Acharyya2001). The BBG belt, located in the southern part of the Sausar Belt (Fig. 1d), is widely composed of quartzofeldspathic gneisses and mafic granulites. This belt witnessed three distinct high-grade metamorphic events (M1, M2 and M3), which are illustrated by looping P–T paths, most likely indicating repeated cycles of slab rollback and advancements of the oceanic crust that was subducting beneath the SIC (Bhowmik et al. Reference Bhowmik, Wilde and Bhandari2011; Bhowmik & Chakraborty, Reference Bhowmik and Chakraborty2017). Monazite and zircon ages obtained for BBG lithologies suggest that the high-grade metamorphic events are bracketed between 1.62 and 1.54 Ga (Bhowmik et al. Reference Bhowmik, Wilde and Bhandari2011, Reference Bhowmik, Wilde, Bhandari and Sarbadhikari2014; Fig. 3). Furthermore, the occurrence of U–Pb zircon ages of 3.5–2.5 Ga reveal that the BBG belt contains vestiges of older reworked crust possibly derived from the Bastar Craton (Ramachandra & Roy, Reference Ramachandra and Roy2001; Bhowmik et al. Reference Bhowmik, Wilde and Bhandari2011). The RKG belt is exposed in the central part of the CITZ, and is dominated by migmatitic gneisses, mafic granulites, cordierite gneisses and garnet-bearing metadolerites (Bhowmik et al. Reference Bhowmik, Pal, Pant and Shome1999). Previous studies have noticed that these rocks exhibit a clockwise P–T trajectory path, with the peak metamorphism at c. 1.04 Ga, followed by a decompression event at c. 0.95 Ga (Bhowmik et al. Reference Bhowmik, Wilde, Bhandari, Pal and Pant2012; Fig. 3). Together, it is evident that the timing of high-grade metamorphism of the RKG belt is distinct from that of the MG and BBG belts.
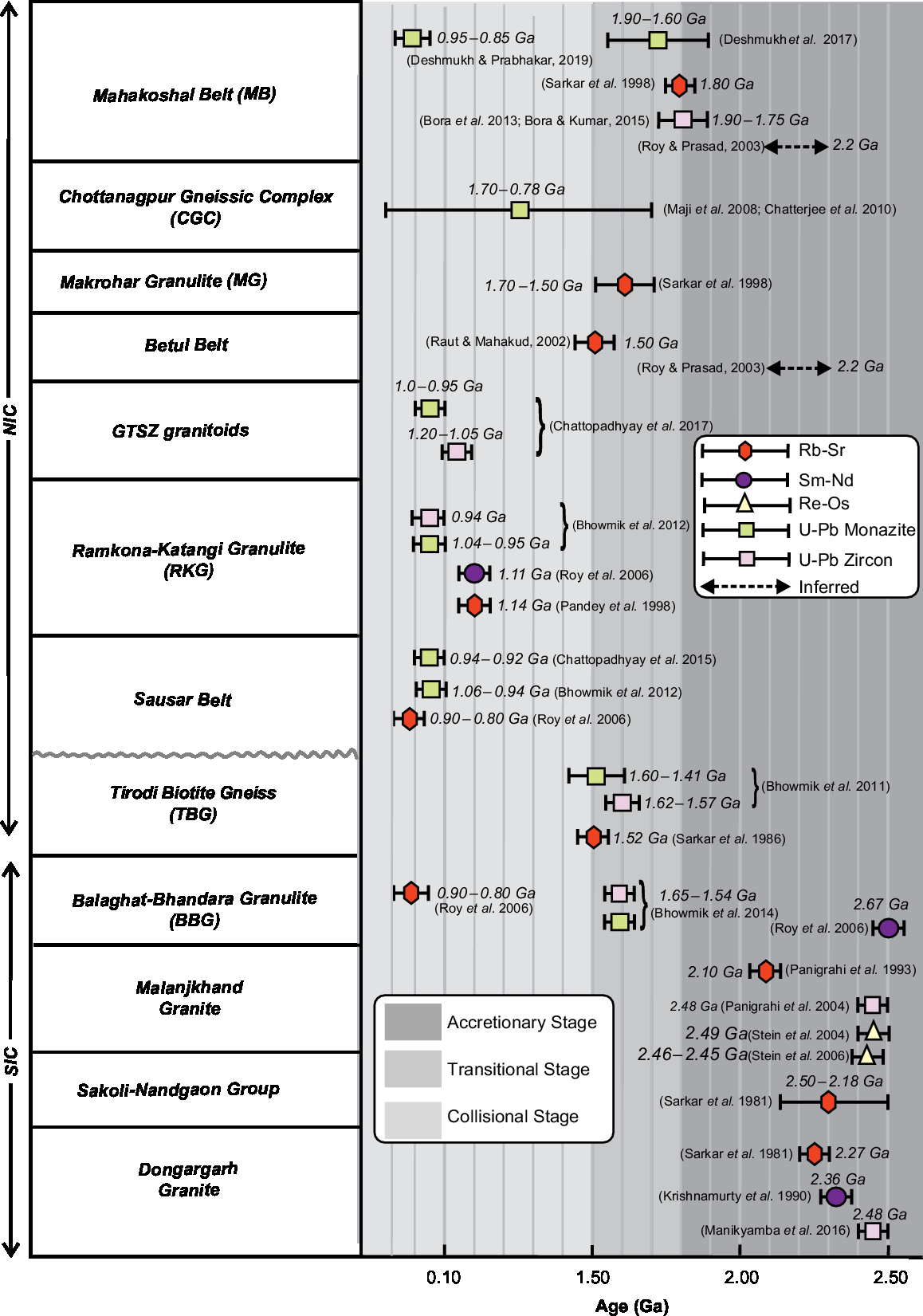
Fig. 3. Summary of available age data, acquired using various geochronological techniques from different domains of the CITZ. Abbreviations for litho-units are in accordance with Figure 1.
2.b. Low- to medium-grade supracrustal belts
The greenschist- to amphibolite-facies supracrustal belts in the CITZ are represented by the Mahakoshal Belt (MB) in the north, the Betul Belt in the central part and the Sausar Belt in the south (Fig. 1d). Spatially, these belts are distributed parallel to the high-grade belts, and other minor lithologies within the CITZ (Fig. 1d). The E–W-trending MB is regarded as the oldest (c. 2.2 Ga) supracrustal belt in the CITZ (Nair et al. Reference Nair, Jain and Yedekar1995; Pandey et al. Reference Pandey, Krishna and Chabria1998), and is composed of phyllites, garnet–staurolite schists, andalusite schists and quartzites. The prominent metamorphic events (M1, M2 and M3) in the belt are dated between 1.9 Ga and 1.6 Ga (Figs 2, 3), where the peak metamorphism (M2) occurred at c. 1.7 Ga (Deshmukh et al. Reference Deshmukh, Prabhakar, Bhattacharya and Madhavan2017; Deshmukh & Prabhakar, Reference Deshmukh and Prabhakar2019). This peak metamorphism likely took place at c. 1.7 Ga, as indicated by the coeval emplacement of granitoids along the southern margin of the MB (Deshmukh & Prabhakar, Reference Deshmukh and Prabhakar2019) and the intrusion of the Jhirgadandi pluton within the MB (Bora et al. Reference Bora, Kumar, Yi, Kim and Lee2013). The Betul Belt, located in the central part of the CITZ, shares tectonic contact with the Gavligarh–Tan Shear Zone (GTSZ) along its southern margin (Fig. 1d), and is characterized by phyllites with intercalated mica schists. Based on early geochronological studies, the upper age of the Betul Belt is constrained at c. 1.50 Ga (Fig. 3; Raut & Mahakud, Reference Raut and Mahakud2002) using Rb–Sr ages of syntectonic granite that are unlikely to represent the age of crystallization. Despite the limited petrological and geochronological data from the Mahakoshal and Betul belts, Roy & Prasad (Reference Roy and Prasad2003) postulated that both the basins opened simultaneously at c. 2.2 Ga, with prolonged sedimentation between c. 2.2 Ga and 1.9 Ga (Fig. 4a). The Sausar supracrustals, along with the surrounding RKG and BBG belts, are together referred to as the Sausar Mobile Belt (SMB) (Roy et al. Reference Roy, Kagami, Yoshida, Roy, Bandyopadhyay, Chattopadhyay, Khan, Huin and Pal2006). Nevertheless, these lithologies, with their variable metamorphic grade, are considered to be associated with the undifferentiated TBG (1.62 Ga) that forms the basement for the Sausar Group of rocks (Bhowmik et al. Reference Bhowmik, Pal, Pant and Shome1999, Reference Bhowmik, Wilde and Bhandari2011). The SMB underwent extensive reworking and amphibolite-facies metamorphism during the Sausar Orogeny at 1.0–0.9 Ga (Fig. 2; Bhowmik et al. Reference Bhowmik, Wilde and Bhandari2011, Reference Bhowmik, Wilde, Bhandari, Pal and Pant2012). Initially, this Grenvillian event was believed to have affected lithologies within the southernmost part of the CITZ, but recent monazite dating indicates a northward extension of the Neoproterozoic tectonic front towards the MB and associated granitoids (Figs 2, 3; Deshmukh et al. Reference Deshmukh, Prabhakar, Bhattacharya and Madhavan2017; Deshmukh & Prabhakar, Reference Deshmukh and Prabhakar2019).

Fig. 4. Schematic diagram (not to scale) illustrating the accretion and evolution of various lithological domains within the CITZ. (a, b) Accretionary stage (2.50–1.80 Ga): Initiation of S-directed subduction system (c. 2.5 Ga) and the development of island-arc magmatism (c. 2.48 Ga) on the SIC, followed by the closing of the Mahakoshal basin (1.90–1.80 Ga; D1 event) with the emplacement of syntectonic granites. (c, d) Transitional stage (1.75–1.55 Ga): Continued deformation within the evolving domains of the CITZ and high-grade metamorphism (BBG) on account of asthenospheric upwelling produced in response to slab break-off (1.65–1.55 Ga) underneath the SIC. (e, f) Collisional stage (1.50–0.85 Ga): Subduction polarity reversal (< 1.55 Ga) leading to the N-directed subduction of the SIC beneath the NIC followed by the development and tightening of ENE–WSW fabric within the CITZ during the Sausar Orogeny (c. 1.0–0.9 Ga). Abbreviations for litho-units are in accordance with Figure 1.
2.c. Magmatic arc sequences in the SIC
The lithological units of the southern cratonic nuclei are dominated by tonalite–trondhjemite–granodiorite (TTG) basement gneisses, volcano-sedimentary supracrustal rocks (viz., Amgaon and Sakoli–Nandgaon groups; Manikyamba et al. Reference Manikyamba, Santosh, Kumar, Rambabu, Tang, Saha, Khelen, Ganguly, Dhanakumar and Rao2016) and associated granitoids (viz., Malanjkhand Granite and Dongargarh Granite; Pandit & Panigrahi, Reference Pandit and Panigrahi2012 and references therein). The Malanjkhand Granite, located along the northern margin of the Bastar Craton, is known for a giant porphyry-style Cu–Mo–Au mineral deposit, and is considered to be associated with the subduction of the NIC beneath the SIC (Yedekar et al. Reference Yedekar, Jain, Nair and Dutta1990). Re–Os dating of molybdenite samples from the Malanjkhand Granite yielded a crystallization age of 2490 ± 8 Ma (Stein et al. Reference Stein, Hannah, Zimmerman, Markey, Sarkar and Pal2004). Stein et al. (Reference Stein, Hannah, Zimmerman and Markey2006) suggested that the deformation in the central parts of the Malanjkhand Granite ceased before c. 2465 Ma, given the occurrence of undeformed molybdenite spindles in the Devgaon area. However, the authors emphasized that the precipitation and mineralization in the northwestern parts of the batholith continued until c. 2450 Ma owing to the intense metamorphic reworking. Based on geochemical and isotopic studies, the Malanjkhand Granite is classified as calc-alkaline, metaluminous (I-type) to peraluminous (S-type) (Arya et al. Reference Arya, Kumar, Gupta, Kumar, Panwar, Deepti and Khan2019 and references therein), with an island-arc affinity (Yedekar et al. Reference Yedekar, Jain, Nair and Dutta1990; Stein et al. Reference Stein, Hannah, Zimmerman, Markey, Sarkar and Pal2004). This interpretation is supported by the presence of microgranular enclaves in the Malanjkhand Granite, produced owing to the mixing and mingling of coeval mafic and felsic magmas (Kumar & Rino, Reference Kumar and Rino2006). All these observations indicate that the Malanjkhand Granite was emplaced along an active continental margin and eventually embedded along the northern margin of the SIC during the early development of the CITZ.
The Dongargarh Granite, located to the south of the Malanjkhand Granite, is typically associated with bimodal volcanic rocks of the Sakoli–Nandgaon Group. Geochemically, bimodal volcanic rocks are interpreted to have developed in an island-arc/back-arc tectonic setting, whereas the granitoids are classified as potassic and within-plate A-type granites (Pandit & Panigrahi, Reference Pandit and Panigrahi2012; Manikyamba et al. Reference Manikyamba, Santosh, Kumar, Rambabu, Tang, Saha, Khelen, Ganguly, Dhanakumar and Rao2016). U–Pb zircon dating of the Dongargarh Granite yielded a crystallization age of 2.48 Ga (Manikyamba et al. Reference Manikyamba, Santosh, Kumar, Rambabu, Tang, Saha, Khelen, Ganguly, Dhanakumar and Rao2016) while earlier studies inferred a Rb–Sr age of c. 2.27 Ga (Sarkar et al. Reference Sarkar, Gopalan and Trivedi1981) and Sm–Nd age of c. 2.36 Ga (Krishnamurthy et al. Reference Krishnamurthy, Sinha, Rai, Seth and Singh1990) (Fig. 3). Furthermore, zircons obtained from basaltic and rhyolitic samples (volcanic units) confirm the age of crystallization at 2471 ± 7 Ma and 2479 ± 15 Ma (Manikyamba et al. Reference Manikyamba, Santosh, Kumar, Rambabu, Tang, Saha, Khelen, Ganguly, Dhanakumar and Rao2016). These zircons display variable εHf(t) values of −10.0 to −6.4 (model ages, TDMC: 3.38–3.60 Ga) and −8.8 to −4.3 (model ages, TDMC: 3.27–3.54 Ga) for volcanic units and granitoids, respectively, indicating a Palaeoarchaean crustal source (Manikyamba et al. Reference Manikyamba, Santosh, Kumar, Rambabu, Tang, Saha, Khelen, Ganguly, Dhanakumar and Rao2016), likely in a continental margin setting. On the basis of bulk-rock geochemistry and Hf–Nd systematics, the Dongargarh volcanic rocks represent an Andean-type magmatic arc developed along the northern part of the Bastar Craton (Khanna et al. Reference Khanna, Bizimis, Barbeau, Krishna and Sai2018).
In summary, the northern margin of the SIC witnessed abundant magmatism during early Palaeoproterozoic times (c. 2.50–2.45 Ga), which was dominated by contemporaneously evolved volcanogenic basalts and rhyolites (Sakoli–Nandgaon Group), and granite plutons (viz. Malanjkhand and Dongargarh granites). Notably, the Malanjkhand Granite is represented by a porphyry Cu–Mo–Au deposit, which is typically associated with arc-related subduction zone magmas (cf. Richards, Reference Richards2003). In other words, according to geochemical–isotopic characteristics, various magmatic rocks were emplaced in a subduction–collision-type tectonic setting (Stein et al. Reference Stein, Hannah, Zimmerman, Markey, Sarkar and Pal2004; Manikyamba et al. Reference Manikyamba, Santosh, Kumar, Rambabu, Tang, Saha, Khelen, Ganguly, Dhanakumar and Rao2016), and their crystallization ages provide a limit for attenuation of subduction-related magmatism in the SIC.
3. Existing tectonic models
Tectonic conditions for the evolution of the CITZ have been discussed and debated for nearly three decades (Yedekar et al. Reference Yedekar, Jain, Nair and Dutta1990; Acharyya, Reference Acharyya2003; Roy & Prasad, Reference Roy and Prasad2003; Bhowmik & Chakraborty, Reference Bhowmik and Chakraborty2017). Among these various models, Yedekar et al. (Reference Yedekar, Jain, Nair and Dutta1990) proposed the first hypothesis, which merged datasets from structural, geochemical and deep continental studies. This model advocates S-directed subduction of the NIC underneath the SIC along the CIS located south of the BBG (Yedekar et al. Reference Yedekar, Reddy, Rao, Varma and Mahadevan2000; Reference Yedekar, Karmalkar and Pawar2003). This early Mesoproterozoic suture zone, extending over 500 km along-strike, is represented by mylonites and phyllonites (Yedekar et al. Reference Yedekar, Reddy, Rao, Varma and Mahadevan2000, Reference Yedekar, Karmalkar and Pawar2003). Based on lithological associations and structural differences, the migmatites and gneisses to the north and south of the CIS are designated as the Tirodi and Amgaon gneisses, respectively (Jain et al. Reference Jain, Yedekar and Nair1991). The Tirodi gneisses and the granulite belts represent the high-grade gneissic terrane of the NIC, whereas the Malanjkhand Granite, Dongargarh Granite and Sakoli–Nandgaon supracrustals represent the island-arc sequences on the overriding SIC (Yedekar et al. Reference Yedekar, Jain, Nair and Dutta1990; Jain et al. Reference Jain, Yedekar and Nair1991, Reference Jain, Nair and Yedekar1995). In this setting, the BBG is interpreted to represent remnants of exhumed oceanic crust (Yedekar et al. Reference Yedekar, Jain, Nair and Dutta1990). However, this model was criticized as it failed to provide evidence for the presence of an ophiolite sequence, deep-sea sediments and high-pressure metamorphic rocks (Bandyopadhyay et al. Reference Bandyopadhyay, Roy and Huin1995). Furthermore, recent geochemical (Ramachandra & Roy, Reference Ramachandra and Roy2001) and geochronological (Bhowmik et al. Reference Bhowmik, Wilde, Bhandari and Sarbadhikari2014) studies disproved the status of the BBG as exhumed oceanic crust, and subsequently moved the possible zone of accretion (i.e. CIS) to the north of the BBG (Bhowmik, Reference Bhowmik2019).
Acharyya (Reference Acharyya2003) proposed a tectonic model in which the Indian subcontinent is divided into southern and northern crustal provinces along the CITZ. The model assumes the MB as a major lithological domain along the northern fringe of the CITZ that possibly corresponds to a continental rift zone. The closure of the Mahakoshal basin owing to S-directed subduction marks the earliest tectonic event within the CITZ, consequently producing calc-alkaline magmatism along the southern margin of the belt (1.8–1.7 Ga). On the other hand, the southern part of the CITZ is predominated by the SMB along with the Chottanagpur Gneissic Complex and Betul supracrustal belt. These crustal domains evolved during two major tectonic events, the collisional event at 1.6–1.5 Ga and the Sausar Orogeny (1.0–0.9 Ga). Although this model supports the S-directed subduction of the NIC, it fails to delineate and demonstrate the presence of island-arc systems formed during the subduction process.
In a diametrically opposite scenario, Roy & Prasad (Reference Roy and Prasad2003) preferred the N-directed subduction of the SIC lithosphere beneath the NIC. This model emphasizes the opposite sense in the subduction direction based on northward younging of metasedimentary sequences in the Sausar basin, suggesting the derivation of Sausar sediments from the Bastar Craton. This interpretation is consistent with the structural vergence of the Sausar basin, where the proposed hinterland is located in the northern part of the basin. The authors demonstrated that the final closure of the subduction between the NIC and SIC occurred at 1.5 Ga and resulted in the formation of the RKG belt in the vicinity of the accretionary zone. Despite these observations, N-directed subduction cannot explain the presence of island-arc-related magmatic sequences on the SIC and the corresponding absence of those features within the NIC (Yedekar et al. Reference Yedekar, Jain, Nair and Dutta1990; Bora, Reference Bora and Kumar2015).
Recently, Bhowmik & Chakraborty (Reference Bhowmik and Chakraborty2017) interpreted the subduction settings along the southern margin of the CITZ based on metamorphic evolution, diffusion kinetics and geochronological results of high-grade rocks in the BBG, including the development of the TBG. This study applies diffusion chronometry to reconstruct the burial and exhumation rates of short-lived metamorphic episodes, which are critical in illustrating the tectonic style for a single orogenic process. Considering the various short-lived metamorphic events in the BBG (viz., M1: 1.62–1.59 Ga; M2: 1.57 Ga; M3: 1.56–1.54 Ga), the authors attempted to explain the predominance of lithospheric extension versus compression during multiple cycles of slab rollback and advancement. Furthermore, the BBG is assumed to represent the leading edge of the SIC on account of its Palaeoarchaean ages, while the position of the CIS has been relocated to the north of the BBG based on contrasting protoliths for the high-grade rocks on either side of the shear zone (Bhowmik et al. Reference Bhowmik, Wilde and Bhandari2011; Bhowmik, Reference Bhowmik2019).
4. Discussion
4.a. Drawbacks of existing models and scope for new hypothesis
Based on the analysis of the tectonic models discussed above, we infer that the models of Yedekar et al. (Reference Yedekar, Jain, Nair and Dutta1990), Acharyya (Reference Acharyya2003) and Roy & Prasad (Reference Roy and Prasad2003) are mostly based on field observations and provenance studies while giving little weight to geophysical evidence. On the other hand, the observations of Bhowmik & Chakraborty (Reference Bhowmik and Chakraborty2017) are restricted to the domains around the CIS (i.e. BBG and TBG) while ignoring the presence of subduction-related arc-magmatism within the SIC. Nevertheless, the major controversies in existing models revolve around the direction of subduction and the precise constraints on the timing of accretion between the NIC and the SIC.
Recently, deep seismic reflection studies have identified the presence of two distinct fabric domains with opposing dip directions to the north and south of the CIS (Mall et al. Reference Mall, Reddy and Mooney2008; Mandal et al. Reference Mandal, Sen and Vijaya Rao2013), which allows re-evaluation of the tectonic regime within the CITZ. The seismic reflection data obtained by Mandal et al. (Reference Mandal, Sen and Vijaya Rao2013) have been processed using the common reflection surface (CRS) method. The results obtained using the CRS technique are of superior quality compared to other conventional techniques (e.g. common mid-point and common reflection point methods), and yield valuable information on deep crustal structures of Precambrian shield areas across the globe (cf. Mandal et al. Reference Mandal, Sen, Vaidya and Mann2014, Reference Mandal, Rao, Sarkar, Bhaskar Rao, Raju, Karuppannan and Sen2017 and references therein). According to Mandal et al. (Reference Mandal, Sen and Vijaya Rao2013), the reflection from 16.0 s two-way time (TWT) indicates the Moho at a depth of 48 km in the vicinity of the CIS. The deep continental geological features identified by Mandal et al. (Reference Mandal, Sen and Vijaya Rao2013) and available seismic characteristics (Mall et al. Reference Mall, Reddy and Mooney2008; Naganjaneyulu & Santosh, Reference Naganjaneyulu and Santosh2010) across the CIS are attributed to the collision between different proto-continents. Therefore, the imaged crustal structures acquired with the CRS method not only represent the upper crustal features but also outline seismic patterns present up to the Moho boundary interface.
In this context, and to improve our understanding of the convergence between the SIC and NIC, it is necessary to integrate geological evidence with inferences made by geophysical studies. The present model suggests that the geological setting of the CITZ is governed by three distinct stages: an accretionary stage (2.5–1.8 Ga), transitional stage (1.75–1.55 Ga) and collisional stage (1.50–0.85 Ga).
4.b. Accretionary stage (2.5–1.8 Ga): formation and deformation of Palaeoproterozoic magmatic arc
In the present model, the Sakoli–Nandgaon volcanic rocks and associated Malanjkhand and Dongargarh granites are together termed as a reworked magmatic arc of the Bastar Craton (Yedekar et al. Reference Yedekar, Jain, Nair and Dutta1990; Stein et al. Reference Stein, Hannah, Zimmerman, Markey, Sarkar and Pal2004). It is postulated that the early tectonic cycle (c. 2.5 Ga; Stein et al. Reference Stein, Hannah, Zimmerman, Markey, Sarkar and Pal2004) within the CITZ initiated with the subduction of the oceanic crust attached to the NIC beneath the SIC (Fig. 4a, b). This southward polarity of the subduction is consistent with the presence of calc-alkaline type Malanjkhand Granite with giant porphyry Cu–Mo–Au deposits (c. 2.5 Ga; Stein et al. Reference Stein, Hannah, Zimmerman, Markey, Sarkar and Pal2004, Reference Stein, Hannah, Zimmerman and Markey2006) and bimodal volcanic rocks of the Sakoli–Nandgaon Group including the Dongargarh Granite (c. 2.5 Ga; Manikyamba et al. Reference Manikyamba, Santosh, Kumar, Rambabu, Tang, Saha, Khelen, Ganguly, Dhanakumar and Rao2016 and references therein). These geological observations suggest the presence of a remnant magmatic arc that formed along the northernmost portion of the SIC.
In contrast, Bhowmik & Roy (Reference Bhowmik and Roy2003) argued that the characteristic N–S-trending fabric in the Malanjkhand Granite is discordant with the ENE–WSW-striking CITZ fabric, and therefore this calc-alkaline magmatism cannot represent a product of the subduction between the SIC and NIC. Recent microstructural studies and anisotropy of magnetic susceptibility data, however, suggest syntectonic fabric development in the Malanjkhand Granite (Majumder & Mamtani, Reference Majumder and Mamtani2009). The authors concluded the presence of pervasive magmatic foliation that was syntectonic with the evolution of the CITZ and the emplacement of the Malanjkhand Granite. This N–S-trending synmagmatic fabric reoriented to a NE–SW-directed fabric, proximal to the CIS, synchronous with WNW–ESE-directed convergence between the NIC and SIC in a transpressional regime (Majumder & Mamtani, Reference Majumder and Mamtani2009). Furthermore, geochemical and isotopic data from the Kotri–Dongargarh belt, located south of the Malanjkhand Granite, show an affinity towards an island-arc setting (Manikyamba et al. Reference Manikyamba, Santosh, Kumar, Rambabu, Tang, Saha, Khelen, Ganguly, Dhanakumar and Rao2016). The above-stated evidence, in addition to the lack of early Palaeoproterozoic subduction-related magmatic activity within the NIC, emphasizes southward subduction of the NIC beneath the SIC (Bora, Reference Bora2015).
Contemporaneously (Fig. 4a), the accretionary phase within the NIC is characterized by the opening of the Mahakoshal and Betul basins at c. 2.2 Ga (inferred age of basin opening; Roy & Prasad, Reference Roy and Prasad2003). As the accretion progressed, the metasedimentary sequences of the MB were overprinted with multiple episodes of deformations (D1 to D3), which represent three distinct metamorphic events (M1 to M3) between 1.90 Ga and 1.60 Ga (Figs 2, 4b–d; Deshmukh et al. Reference Deshmukh, Prabhakar, Bhattacharya and Madhavan2017; Deshmukh & Prabhakar, Reference Deshmukh and Prabhakar2019). These events coincide with the timing of syn- to post-tectonic granite magmatism along the southern and northern margins of the belt, respectively (Bora et al. Reference Bora, Kumar, Yi, Kim and Lee2013; Bora & Kumar, Reference Bora and Kumar2015; Deshmukh & Prabhakar, Reference Deshmukh and Prabhakar2019).
4.c. Transitional stage (1.75–1.55 Ga): slab break-off beneath the SIC and associated slab rollback
The continued accretion and subduction between the NIC and SIC produced mantle melting, accompanied by the formation of the TBG (1.62 Ga; Fig. 4d) at the expense of pre-existing tonalite–granodiorite–granite crust (Bhowmik et al. Reference Bhowmik, Wilde and Bhandari2011; Bhowmik, Reference Bhowmik2019). Subsequently, the accretionary front experienced several cycles of slab advancement and rollback during 1.62–1.54 Ga (Bhowmik et al. Reference Bhowmik, Wilde and Bhandari2011; Bhowmik & Chakraborty, Reference Bhowmik and Chakraborty2017), resulting in the formation of the BBG in association with high-grade metamorphism. This c. 1.65–1.55 Ga metamorphism that affected most of the lithological units probably indicates the final tectonothermal event in the S-directed subduction regime. In particular, outcrops of the BBG have been identified with high-gravity values and corresponding dome-shaped seismic reflection patterns, representing an upward-thrusted block from the lower crust (Mishra et al. Reference Mishra, Singh, Tiwari, Gupta and Rao2000). According to Naganjaneyulu & Santosh (Reference Naganjaneyulu and Santosh2010), such domal structures with metamorphic cores are produced as a consequence of slab break-off. This event, referred to as slab break-off, resulted in the detachment of the downgoing NIC, which successively sank into the deeper mantle (Fig. 4d), thus creating a window for N-directed subduction of the SIC lithosphere (Fig. 4e).
The slab break-off is predicted to have initiated along the CIS, where the SIC began its northward advance (Fig. 4e). The slab break-off process is generally coupled with asthenospheric upwelling, which results in a high heat flow and exhumation rate (Barr & Dahlen, Reference Barr and Dahlen1989; Teng et al. Reference Teng, Lee, Tsai and Hsiao2000). These geological features are preserved within high-grade sequences of the BBG belt, where the thermally uplifted domains were subjected to crustal extension. The period of extension associated with the M2 phase of metamorphism (c. 1.57 Ga; Bhowmik & Chakraborty, Reference Bhowmik and Chakraborty2017) of the BBG domain is representative of the crustal extension experienced by the granulite belt on account of gravitational collapse owing to slab break-off (1.65–1.55 Ga). This slab break-off (1.65–1.55 Ga; Fig. 4d) induced a reversal in the direction of subduction (<1.55 Ga); thus setting the course for a N-directed subduction system (Fig. 4e).
4.d. Collisional stage (1.50–0.85 Ga): subduction polarity reversal, Sausar Orogeny and final amalgamation
The subduction polarity reversal (<1.55 Ga; Fig. 4e) was followed by a period of tectonic quiescence during 1.55–1.20 Ga, which might have been associated with relatively flat-subduction of the SIC lithosphere. Lithological domains around the CIS were subjected to intense contractional deformation owing to this flat-subduction. As a result, the orogen experienced emplacement of syntectonic calc-alkaline granites (1.20–1.05 Ga) along the GTSZ (Chattopadhyay & Khasdeo, Reference Chattopadhyay and Khasdeo2011; Chattopadhyay et al. Reference Chattopadhyay, Chatterjee, Das and Sarkar2017). Subsequently, the CIS reactivated during the Sausar Orogeny at 1.0–0.9 Ga (Bhowmik et al. Reference Bhowmik, Wilde, Bhandari, Pal and Pant2012; Fig. 4f). The intensity of the Sausar event is highest in the vicinity of the CIS where it has resulted in amphibolite-facies metamorphism of the SMB (Roy et al. Reference Roy, Kagami, Yoshida, Roy, Bandyopadhyay, Chattopadhyay, Khan, Huin and Pal2006) and deformation of granitoids along the GTSZ (Chattopadhyay & Khasdeo, Reference Chattopadhyay and Khasdeo2011; Chattopadhyay et al. Reference Chattopadhyay, Chatterjee, Das and Sarkar2017). The effect of this event lessens towards the northern margin of the CITZ, where it causes tightening of the pre-existing fabric (Fig. 2; Deshmukh et al. Reference Deshmukh, Prabhakar, Bhattacharya and Madhavan2017; Deshmukh & Prabhakar, Reference Deshmukh and Prabhakar2019). Further, geophysical evidence suggests that the Sausar Orogeny produced a bivergent reflection fabric in the vicinity of the CIS (Mall et al. Reference Mall, Reddy and Mooney2008; Mandal et al. Reference Mandal, Sen and Vijaya Rao2013), implying a northward vergence for the SIC lithosphere during early Neoproterozoic times.
In conclusion, the slab break-off process proposed underneath the NIC is a consequence of ocean closure and is considered to be the driving mechanism for polarity reversal in subduction zones (Davies & von Blanckenburg, Reference Davies and von Blanckenburg1995; Hildebrand & Bowring, Reference Hildebrand and Bowring1999; Teng et al. Reference Teng, Lee, Tsai and Hsiao2000). Although geophysical exploration of the Earth’s interior is yet to confirm the case in favour or against the slab break-off hypothesis (Garzanti et al. Reference Garzanti, Rade and Malusà2018), the present model seems to provide a plausible explanation for the presence of Palaeoproterozoic island-arc sequences on the SIC while simultaneously incorporating the subsurface features observed across the CIS. Previously documented seismic studies (Mall et al. Reference Mall, Reddy and Mooney2008; Naganjaneyulu & Santosh, Reference Naganjaneyulu and Santosh2010; Mandal et al. Reference Mandal, Sen and Vijaya Rao2013 and references therein) within the CITZ are limited to the Moho boundary, whereas gravity modelling studies are yet to recognize the remnants of detached slab beneath the SIC. Therefore, further studies using advanced geophysical techniques (e.g. seismic tomography) are awaited to delineate the presence of a detached crustal segment of the NIC underneath the SIC.
5. Conclusions
(a) The Palaeoproterozoic evolution of the CITZ is characterized by island-arc magmatism (c. 2.5–2.4 Ga) in the Bastar Craton (i.e. Malanjkhand Granite, Dongargarh Granite and Sakoli–Nandgaon volcanic rocks) implying S-directed subduction of the NIC oceanic crust beneath the SIC at c. 2.5 Ga.
(b) The S-directed subduction and deformation process continued until slab break-off beneath the SIC at c. 1.65–1.55 Ga. The protracted accretion during this period (c. 2.50–1.55 Ga) was dominated by the following tectonic events: (i) emplacement and deformation of felsic and mafic suites on the SIC, (ii) opening of rift basins followed by sedimentation and deformation (i.e. Mahakoshal and Betul) on the NIC, (iii) evolution of high-grade crystalline rocks such as Tirodi Biotite Gneiss, Makrohar and Balaghat–Bhandara granulites, and (vi) activation of the ENE–WSW-trending Son-Narmada North Fault and Son-Narmada South Fault on the northern margin and CIS along the southern margin, respectively.
(c) The slab break-off process induced polarity reversal that resulted in the flipping of the subduction direction (<1.55 Ga). The detached segment of the NIC created an opening for the lateral movement of the SIC, eventually setting the course for N-directed subduction. This event coincides with the reworking of the BBG unit, which was subjected to crustal extension as a result of slab break-off. The tectonic events that occurred at this stage (c. 1.50–1.10 Ga) are characterized by the 1.20 Ga granite magmatism along the GTSZ and the formation of the RKG.
(d) The final stage of accretion (c. 1.00–0.85 Ga) is dominated by the Sausar Orogeny, which resulted in the development of an ENE–WSW-trending fabric coupled with reworking along the southern margin (i.e. SMB, GTSZ) of the CITZ. The effect of this event diminishes towards the northern margin, where it is manifested by the tightening of the pre-existing fabric.
Acknowledgements
This study was financially supported by the IRCC project (13IRCCSG025) of the Indian Institute of Bombay, India. TD acknowledges the financial support provided by the CSIR research fellowship (File No: 09/087(0813)/2015-EMR-I). We acknowledge the Science and Engineering Research Board (Govt. of India) funded EPMA national facility in the Department of Earth Sciences at IIT Bombay. The comments by Dr. Holly Stein and two anonymous reviewers considerably improved the clarity and presentation of the manuscript. Editorial handling of the manuscript by Prof. Oliver Lacombe is gratefully appreciated. We acknowledge the discussions with G. Mohan, M. Radhakrishna and B. Sekhar on the possible interpretations of various geophysical data. We also thank P.U. Naveen for his help with interpretation of gravity anomaly measurements from Central India.