Book contents
- Genome Editing and Engineering
- Genome Editing and Engineering
- Copyright page
- Dedication
- Contents
- Contributors
- Foreword
- Preface
- Part I Biology of Endonucleases (Zinc-Finger Nuclease, TALENs and CRISPRs) and Regulatory Networks
- Part II Genome Editing in Model Organisms
- Part III Technology Development and Screening
- Part IV Genome Editing in Stem Cells and Regenerative Biology
- Part V Genome Editing in Disease Biology
- Part VI Legal (Intellectual Property) and Bioethical Issues of Genome Editing
- Index
- Plate Section (PDF Only)
- References
Part III - Technology Development and Screening
Published online by Cambridge University Press: 30 July 2018
- Genome Editing and Engineering
- Genome Editing and Engineering
- Copyright page
- Dedication
- Contents
- Contributors
- Foreword
- Preface
- Part I Biology of Endonucleases (Zinc-Finger Nuclease, TALENs and CRISPRs) and Regulatory Networks
- Part II Genome Editing in Model Organisms
- Part III Technology Development and Screening
- Part IV Genome Editing in Stem Cells and Regenerative Biology
- Part V Genome Editing in Disease Biology
- Part VI Legal (Intellectual Property) and Bioethical Issues of Genome Editing
- Index
- Plate Section (PDF Only)
- References
Summary
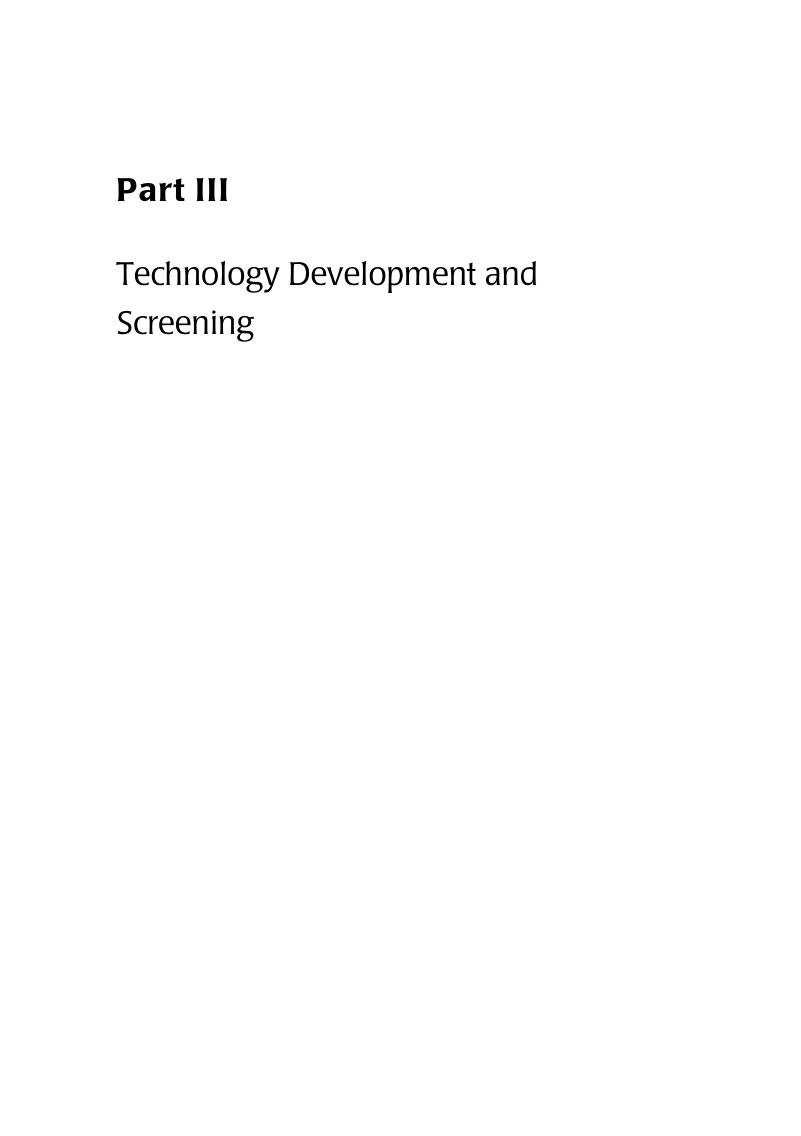
- Type
- Chapter
- Information
- Genome Editing and EngineeringFrom TALENs, ZFNs and CRISPRs to Molecular Surgery, pp. 163 - 234Publisher: Cambridge University PressPrint publication year: 2018