Macronutrient intake represents a potent modulator of acute exercise responses and chronic adaptations, exerting effects on resting metabolism, fuel utilisation during exercise, acute cell signalling and gene expression, and ultimately phenotype( Reference Hawley, Burke and Phillips 1 , Reference Hawley, Tipton and Millard-Stafford 2 ). Carbohydrate (CHO) serves as a key substrate for exercise, as well as mediates multiple molecular responses to endurance training( Reference Hawley, Burke and Phillips 1 – Reference Bartlett, Hawley and Morton 3 ). It is well understood that high daily CHO intake is advantageous for endurance training, especially that of intense nature( Reference Achten, Halson and Moseley 4 , Reference Bergstrom, Hermansen and Hultman 5 ); however, specific recommendations of daily CHO have yet to be established for resistance-based exercise( Reference Campbell 6 ). Moreover, the effects of CHO on the cellular responses to resistance exercise have yet to be fully characterised. The lack of empirical consensus on CHO intake and resistance exercise has resulted in recommendations largely stemming from endurance exercise and/or anecdotally based practices. This is problematic considering that resistance exercise often accounts for a substantial portion of athletes’ training programmes and represents a highly practiced training mode within the recreational athletic community. This apparent shortage of evidence may lead an athlete or practitioner to unwarranted dietary practices, hindering training quality, adaptation and competitive performance.
Furthermore, the topic of CHO intake and exercise performance has become one of polarising nature. Low-CHO diets drawing inspiration from the Atkins Diet, such as the Paleolithic or ‘Paleo’ Diet, Zone Diet and ketogenic diets, first garnered popularity within the general population for weight management and general health purposes; however, such diets have found their way into the domain of exercise and sport nutrition( Reference Wilson 7 – Reference Sisson and Kearns 12 ) prior to their efficacy being established. The current climate in exercise and sport nutrition, particularly that in the general public and media, is one that seemingly creates a dichotomous view, where one is either ‘anti-high CHO’ or ‘anti-low CHO’. Some from the scientific community are currently strong proponents of these movements( Reference Noakes, Volek and Phinney 9 – Reference Volek and Phinney 11 ). However, CHO need for training and performance is one of nuance and exists on a continuous spectrum, varying frequently while considering the athlete’s mode of training, as well as the corresponding intensity and volume and inter-individual variability( Reference Helms, Aragon and Fitschen 13 – Reference Goedecke, St Clair Gibson and Grobler 15 ). Most literature examining low-CHO diets has been focused on endurance exercise( Reference Noakes, Volek and Phinney 9 , Reference Volek, Noakes and Phinney 10 , Reference Burke and Hawley 16 – Reference Burke 18 ). It is worth noting that despite a lack of evidence demonstrating an ergogenic benefit( Reference Phinney, Bistrian and Evans 19 – Reference Zajac, Poprzecki and Maszczyk 22 ) in addition to literature suggesting an increased risk of impaired high-intensity exercise capacity( Reference Burke and Hawley 16 , Reference Burke 18 ), the appeal of a low-CHO diet for endurance performance has not subsided, attesting to the fervour surrounding this topic (the reader is referred to a review by Burke( Reference Burke 18 ) for a discussion of this topic).
Resistance-based exercise is metabolically distinct from endurance training and elicits a broadly different cascade of cellular events which underlie adaptation( Reference Hawley, Hargreaves and Joyner 23 ). Resistance exercise outcomes are primarily instigated by mechanically based stress( Reference Watson and Baar 24 , Reference Marcotte, West and Baar 25 ) rather than metabolic and energetic challenges as elicited by endurance exercise( Reference Hawley, Hargreaves and Joyner 23 ). Therefore, CHO intake will likely influence adaptation to resistance-based exercise in a dissimilar manner compared with endurance exercise, although only a modest amount of literature exists to date pertaining to this question. Furthermore, CHO recommendations exclusively for resistance-based exercise are scarce, and literature addressing exercise nutrition oftentimes does not make any specific recommendations( Reference Thomas, Erdman and Burke 26 , Reference Kreider, Wilborn and Taylor 27 ). Typical recommendations suggest a moderately high CHO intake in the range of 3–7 g/kg per d for resistance exercise trainees( Reference Slater and Phillips 14 , Reference Lambert, Frank and Evans 28 ); however, evidence appearing to challenge this paradigm exists( Reference Camera, West and Burd 29 – Reference Roberts, Holland and Kephart 34 ). Low-CHO diets may not be as detrimental as typically espoused, as investigations have demonstrated that CHO intakes less than the moderately high recommendation does not hinder acute resistance exercise performance( Reference Mitchell, DiLauro and Pizza 30 , Reference Sawyer, Wood and Davidson 32 ) or the subsequent cellular responses responsible for adaptation, including translation initiation and myofibrillar protein synthesis( Reference Camera, West and Burd 29 , Reference Creer, Gallagher and Slivka 31 ).
Scarce recommendations and recent findings call to question the actual CHO need for resistance-based exercise. This review will discuss the influence of CHO intake on resistance-based exercise using literature pertaining to acute resistance exercise performance and training outcomes. In addition, the underlying molecular responses to exercise and nutrient status will be discussed, including the influence of CHO on key cellular signalling pathways (e.g. 5' AMP-activated protein kinase (AMPK), Akt, mammalian target of rapamycin complex 1 (mTORC1)), as well as the implications on training-mediated adaptations.
Literature review
A review of the available literature was conducted using PubMed, Medline and SPORTDiscus databases, which were each searched for relevant keywords and phrases, alone or in combination, including carbohydrate, resistance exercise, glycogen, sports nutrition, performance, protein synthesis, supplementation, mTOR, AMPK, protein degradation, insulin, anabolism and so on. Given the nature of this topic, which includes elements of well-studied topics (i.e. CHO and endurance exercise, supplementation and protein synthesis, interaction of mTOR and AMPK and so on), substantial individual selection from high-return search results was required despite extensive manipulation with Boolean commands. Only studies published in an English-language refereed journal were included.
Current recommendations for carbohydrate intake
Individual macronutrient needs must be specific to the mode, volume, intensity and frequency of training, as well as accounting for inter-individual variability( Reference Helms, Aragon and Fitschen 13 – Reference Goedecke, St Clair Gibson and Grobler 15 ). However, ranges of macronutrient recommendations for exercise do exist based on experimental observation, providing guidelines to assist in individualising nutrient intake( Reference Slater and Phillips 14 , Reference Thomas, Erdman and Burke 26 , Reference Kreider, Wilborn and Taylor 27 ). With regard to CHO, the American College of Sports Medicine, American Dietetic Association and the Dieticians of Canada( Reference Thomas, Erdman and Burke 26 ) recommend CHO targets ranging from 3 to 5 g/d for low-intensity or skill-based activities to 8–12 g/kg per d for very high training demands. The International Society of Sports Nutrition( Reference Kreider, Wilborn and Taylor 27 ) suggests 5–10 g/kg per d for athletes involved in moderate and high volumes of intense training, and Costill & Hargreaves( Reference Costill and Hargreaves 35 ) recommend 8–10 g/kg per d or 60–70 % of daily energy intake during periods of heavy training. Interestingly, specific recommendations regarding CHO intake and resistance exercise are not made in any of this literature. It is well understood that such CHO-rich diets optimise performance in endurance-based training and competition( Reference Achten, Halson and Moseley 4 , Reference Bergstrom, Hermansen and Hultman 5 ); however, the specific CHO requirements for individuals whose primary mode of training is based on resistance exercise have yet to be conclusively established( Reference Campbell 6 , Reference Helms, Aragon and Fitschen 13 ). An acute bout of resistance exercise can reduce muscle glycogen content by approximately 25–40 %( Reference Robergs, Pearson and Costill 36 , Reference Camera, Edge and Short 37 ), and thus it may be suggested that adequate daily CHO is warranted for glycogen repletion during periods of regular training. Slater & Phillips( Reference Slater and Phillips 14 ) recommend a moderate 3–5 g/kg per d for strength and power athletes and 4–7 g/kg per d for bodybuilders. Lambert et al.( Reference Lambert, Frank and Evans 28 ) recommends a CHO intake of 5–6 g/kg per d or 55–60 % of daily energy intake for bodybuilders. However, and of particular note, it has been observed that daily CHO intake considerably less than such recommendations does not impair resistance-based exercise performance( Reference Mitchell, DiLauro and Pizza 30 , Reference Sawyer, Wood and Davidson 32 , Reference Dipla, Makri and Zafeiridis 33 , Reference Paoli, Grimaldi and D’Agostino 38 – Reference Van Zant, Conway and Seale 40 ) or hinder the requisite post-exercise cellular signalling responses for adaptation( Reference Camera, West and Burd 29 , Reference Creer, Gallagher and Slivka 31 , Reference Churchley, Coffey and Pedersen 41 , Reference Camera, Hawley and Coffey 42 ).
Carbohydrate and resistance-based exercise performance
The production and maintenance of muscular force during resistance exercise relies heavily on energy produced from the breakdown of phosphocreatine and muscle glycogen. Consequently, it may be argued that daily intake of CHO must be adequate to replete muscle glycogen stores( Reference Haff and Whitley 43 ). However, if this is indeed the case (data presented in later sections may suggest otherwise), what constitutes ‘adequate’ for resistance-based exercise? While glycogen serves as a substrate during resistance training, the total energy expenditure of strength athletes is less than that of mixed sport and endurance athletes( Reference Helms, Aragon and Fitschen 13 ), and experimental evidence has yet to confirm a minimum threshold of daily CHO intake for resistance trainees. Although Leveritt & Abernathy( Reference Leveritt and Abernathy 39 ) reported a decrease in the number of back squat repetitions to failure following a low-CHO diet consisting of 1·26 g/kg per d (111·9 g/d; 19·3 % of energy intake) for 2 d in recreationally active subjects, the following investigations have demonstrated that modest, and even minimal, amounts of CHO may maintain resistance-based exercise performance (Table 1). Mitchell et al.( Reference Mitchell, DiLauro and Pizza 30 ) found that volumes (load×repetitions) of back squats, leg press and knee extensions were not compromised following 48 h of 0·4 g/kg per d CHO (31·6 g/d; 4·1 % of energy intake) compared with a high CHO intake of 7·7 g/kg per d (642·6 g/d; 80·2 % of energy intake) in recreationally trained males. In a crossover study, Hatfield et al.( Reference Hatfield, Kraemer and Volek 44 ) investigated the effect of 4 d of CHO-loading on resistance training performance. Eight recreationally resistance-trained men consumed a diet of 50 and 80 % CHO and performed four sets of twelve repetitions of maximal effort squat jumps at 30 % one repetition maximum (1RM) before and after each CHO intervention. There were no significant differences in peak power, mean power or total work accomplished in repetitive jump performance at 30 % of 1RM between diets comprised of 50 and 80 % CHO, respectively. In another crossover study by Dipla et al.( Reference Dipla, Makri and Zafeiridis 33 ), ten recreationally active women consumed a control diet consisting of 55 % CHO, 15 % protein and 30 % fat or a moderately low-CHO diet of 30 % CHO, 40 % protein and 30 % fat for 1 week each and performed tests of upper- and lower-body strength. No differences were found between dietary interventions in isometric handgrip strength, handgrip endurance, peak torque of isokinetic knee extension or knee flexion. In addition, Sawyer et al.( Reference Sawyer, Wood and Davidson 32 ) imposed a hypoenergetic very-low-CHO (30·77 g/d; 5·4 % of daily kcal intake) diet on thirty-one trained individuals (sixteen men and fifteen women) for 7 d following 7 d of mixed diet (265·40 g/kg; 40·7 % of daily kcal intake) and measured pre- and post-intervention handgrip strength, vertical jump height, 1RM bench press, 1RM squat, upper-body power output and Wingate peak power. 1RM squat, vertical jump height and handgrip strength increased following the CHO-restricted diet, whereas bench press strength, upper-body power and Wingate peak power output were maintained. Van Zant et al.( Reference Van Zant, Conway and Seale 40 ) reported that 3 weeks of a moderate-CHO diet (42 % CHO, 40 % fat and 18 % protein) resulted in no difference in muscular strength or endurance as measured by isokinetic knee extension and flexion, bench press 1RM and repetitions to failure at 80 % 1RM when compared with a high-CHO diet (62 % CHO, 20 % fat and 18 % protein). Similarly, Paoli et al.( Reference Paoli, Grimaldi and D’Agostino 38 ) reported that performance of squat jumps, countermovement jumps, consecutive countermovement jumps, push-ups, reverse grip chin ups and parallel bar dips test were maintained following 30 d of very-low-CHO ketogenic diet (4·5 % CHO, 54·8 % fat and 40·7 % protein) in nine elite gymnasts.
Table 1 A summary of investigations examining carbohydrate (CHO) intake manipulation and acute resistance-based exercise performance

RM, repetition maximum; PVO2, peak cycle ergometer VO2.
In addition to this human work, recent work involving a rodent model offers some of the only data investigating longer-term adaptations to resistance training while ingesting minimal amounts of CHO. Roberts et al.( Reference Roberts, Holland and Kephart 34 ) fed rats a ketogenic diet (10·3 % CHO, 20·2 % protein, 69·5 % fat) or a Western diet (42·7 % CHO, 15·2 % protein, 42·0 % fat) for 6 weeks during a resistance-training model consisting of resistance-loaded voluntary wheel running and measured muscular hypertrophy of the gastrocnemius. Following the 6-week diet and exercise intervention, both groups incurred an increase in myofibrillar protein content; however, no differences were observed between the ketogenic diet and the Western diet( Reference Roberts, Holland and Kephart 34 ).
The maintenance of strength and power performance in response to a short-term CHO-restricted diet (i.e. 2–4 d)( Reference Leveritt and Abernathy 39 , Reference Hatfield, Kraemer and Volek 44 ) may not be unexpected, as this may be an insufficient time table to substantially decrease muscle glycogen stores. A number of the reported studies, however, did not collect skeletal muscle samples to assess intramuscular glycogen levels. In this respect, many of the aforementioned studies may only speculate as to whether the CHO intake manipulations altered muscle glycogen content. Moreover, the true risk of glycogen depletion during regular resistance exercise is unclear. An acute bout of resistance exercise can reduce glycogen concentrations by approximately 25–40 %( Reference Helms, Aragon and Fitschen 13 , Reference Robergs, Pearson and Costill 36 , Reference Camera, Edge and Short 37 , Reference Roy and Tarnopolsky 45 ); however, even when no food or CHO is consumed in the post-exercise period, glycogen may be synthesised at an hourly rate of approximately 1·3–11 mmol/kg wet weight( Reference Pascoe and Gladden 46 ). Robergs et al.( Reference Robergs, Pearson and Costill 36 ) measured muscle glycogen in eight resistance-trained males before, immediately after and 2 h following six sets of six repetitions of leg extension at 70 % 1RM. Subjects were not fed during the 2-h post-exercise period. Immediately post-exercise, glycogen concentrations were 61 % of pre-exercise values. 2 h post-exercise, muscle glycogen concentrations were 79 % of pre-exercise levels. In their review of nutrient timing, Aragon & Schoenfeld( Reference Aragon and Schoenfeld 47 ) suggest that incomplete resynthesis of muscular glycogen would not be a concern aside from the unlikely scenario of exhaustive training bouts of the same musculature occurring in recovery intervals <24 h. Considering that many resistance-training programmes utilise 2–3 d recovery periods between training muscle groups, it is likely that even modest amounts of CHO are capable of replenishing glycogen stores between training bouts of particular musculature.
Nutrient intake, resistance exercise and cell signalling
Macronutrient intake is capable of modulating the acute regulatory processes that underlie cell signalling, gene expression and thus adaptation (Fig. 1)( Reference Hawley, Burke and Phillips 1 ). CHO intake and subsequent intramuscular glycogen concentrations play a role in the energy status and signalling activity of skeletal muscle, namely by influencing the activity of AMPK( Reference Hawley, Burke and Phillips 1 , Reference Hawley, Tipton and Millard-Stafford 2 , Reference Aschenbach, Sakamoto and Goodyear 48 , Reference McBride, Ghilagaber and Nikolaev 49 ). AMPK is a metabolite-sensing kinase that monitors cellular energy status, regulating the activity of catabolic and anabolic pathways, as well as the transcription of several exercise-responsive genes( Reference Hawley, Hargreaves and Joyner 23 , Reference Aschenbach, Sakamoto and Goodyear 48 , Reference Coffey and Hawley 50 – Reference Jorgensen, Richter and Wojtaszewski 52 ). Although AMPK activity is crucial to support muscular contraction( Reference Aschenbach, Sakamoto and Goodyear 48 ), AMPK is capable of exerting an inhibitory effect on the cellular signalling pathways involved in the adaptive responses to resistance-based exercise, namely protein synthesis( Reference Bolster, Crozier and Kimball 53 , Reference Xu, Ji and Yan 54 ). AMPK up-regulates protein degradation pathways including autophagy and the ubiquitin proteasome pathway( Reference Goodman, Mayhew and Hornberger 55 , Reference Sandri 56 ) while serving as a negative regulator of the mTORC1( Reference Xu, Ji and Yan 54 , Reference Dreyer, Fujita and Cadenas 57 , Reference Williamson, Bolster and Kimball 58 ). mTORC1 is a central regulatory kinase involved in cell growth and protein synthesis and is stimulated by amino acids, as well as mechanical loading, such as that elicited by resistance exercise( Reference Watson and Baar 24 , Reference Marcotte, West and Baar 25 ). Within the immediate post-exercise period (0–1 h) following resistance exercise, the intracellular environment transitions from an initial degradation phase to an anabolic phase mediated by decreased AMPK activity and up-regulation of mTORC1 activity and translation initiation( Reference Camera, West and Burd 29 , Reference Roberts, Holland and Kephart 34 , Reference Dreyer, Fujita and Cadenas 57 , Reference Lundberg, Fernandez-Gonzalo and Tesch 59 , Reference Wilkinson, Phillips and Atherton 60 ). AMPK contains glycogen-binding sites( Reference McBride, Ghilagaber and Nikolaev 49 ), leading to the hypothesis that this protein complex may be allosterically modified by muscle glycogen status( Reference Aschenbach, Sakamoto and Goodyear 48 , Reference McBride, Ghilagaber and Nikolaev 49 , Reference McBride and Hardie 61 ). While currently the physiological significance of glycogen-mediated AMPK regulation is unclear( Reference Carling, Mayer and Sanders 62 – Reference Roepstorff, Vistisen and Donsmark 66 ), it has been speculated that very-low-CHO diets, such as ketogenic diets( Reference Paoli, Bianco and Grimaldi 67 ), and low-glycogen concentrations( Reference Hawley, Burke and Phillips 1 , Reference Aschenbach, Sakamoto and Goodyear 48 , Reference Wojtaszewski, MacDonald and Nielsen 63 ) may result in up-regulated AMPK activity and thus may not be recommended for incurring muscular hypertrophy given its inhibitory effects on mTORC1( Reference Xu, Ji and Yan 54 , Reference Dreyer, Fujita and Cadenas 57 ). Roberts et al.( Reference Roberts, Holland and Kephart 34 ) reported no difference in basal αAMPKThr172 phosphorylation, as well as no differences at 90, 180, 270 min post-resistance exercise in rats following 6 weeks of an isoenergetic intake of either a ketogenic diet (10·3 % CHO, 20·2 % protein, 69·5 % fat) or a Western diet (42·7 % CHO, 15·2 % protein, 42·0 % fat). In addition, although commencing endurance exercise with low-glycogen concentrations results in increased AMPK activity when compared with normal concentrations( Reference Bartlett, Hawley and Morton 3 , Reference Wojtaszewski, MacDonald and Nielsen 63 , Reference Yeo, McGee and Carey 68 ), this relationship has yet to be established with resistance exercise. However, current evidence suggests that intramuscular glycogen content does not augment basal AMPK activity( Reference Camera, West and Burd 29 , Reference Steinberg, Watt and McGee 64 , Reference Watt, Steinberg and Chan 65 ).
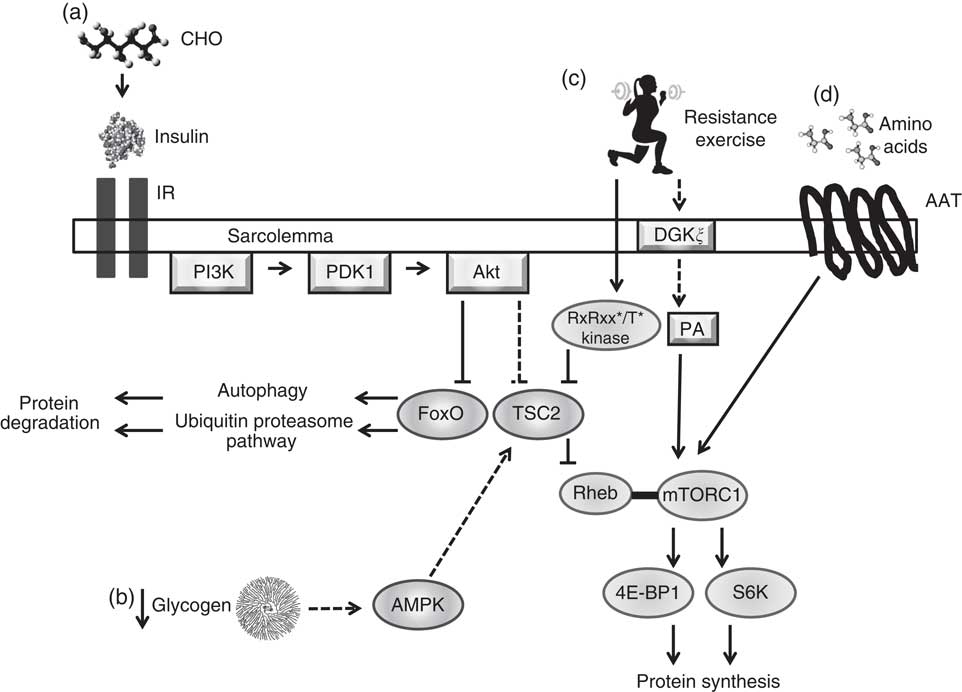
Fig. 1 Simplified illustration of cell signalling pathways associated with protein synthesis and degradation in skeletal muscle resulting from nutrient intake, glycogen concentrations and mechanical loading. (a) Carbohydrate (CHO) ingestion results in the secretion of insulin from the pancreas into the blood, which binds to insulin receptors (IR) on the sarcolemma, activating the phosphatidylinositol-3-OH kinase (PI3K)-Akt pathway. Akt inhibits forkhead box O (FoxO) activity by promoting its exportation from the nucleus into the cytoplasm and inducing its degradation, inhibiting protein degradation systems autophagy and the ubiquitin proteasome pathway. Akt removes tuberous sclerosis complex 2 (TSC2) from the lysosomal membrane, allowing for mammalian target of rapamycin complex 1 (mTORC1) to interact with its activator Ras homologue enriched in bran (Rheb) at the lysosome. Activation of mTORC1 results in increased activity of ribosomal kinases S6 (S6k; p70S6K, p90S6K) and inhibition of eukaryotic initiation factor 4E-binding protein (4E-BP1), leading to protein synthesis. , Physiological concentrations of insulin have not been shown to increase protein synthesis in human muscle. (b) Low muscle glycogen levels may increase 5' AMP-activated protein kinase (AMPK) activity, leading to an enhanced activity of TSC2 and inhibition of mTORC1 activity.
, Unestablished effect of glycogen concentrations on AMPK in human skeletal muscle at rest or during and after resistance exercise; inhibitory effect of AMPK on mTORC1 may not impair mTORC1 activity in human skeletal muscle following resistance exercise or amino acid feeding. (c) Heavy mechanical loading (i.e. resistance exercise) activates a currently unknown kinase, which phosphorylates TSC2 within a RxRxx*/T* motif resulting in its translocation from the lysosome, allowing mTORC1 to bind with Rheb. Phosphatidic acid (PA) activates mTORC1, possibly by direct binding to the 12-kDa FK506-binding protein (FKBP12)-rapamycin-binding domain.
, Increased PA is likely mediated by diacylglycerol kinase ξ (DGKξ) activity. (d) Amino acid transporters (AAT) uptake amino acids into the sarcoplasm. An increase in intracellular amino acid levels facilitate translocation of mTORC1 to the lysosomal surface mediated by the Rag family of G-proteins where it can interact with Rheb.
,
, Stimulatory response;
,
, inhibitory response.
An in vitro study by McBride et al.( Reference McBride, Ghilagaber and Nikolaev 49 ) demonstrated that treatment of oligosaccharides allosterically inhibited AMPK; however, an investigation by Polekhina et al.( Reference Polekhina, Gupta and Michell 69 ) found that glycogen did not inhibit purified rat liver AMPK. Moreover, human studies have yielded conflicting results regarding the influence of glycogen content and AMPK activity at rest. Wojtaszewski et al.( Reference Wojtaszewski, MacDonald and Nielsen 63 ) reported that a low glycogen concentration of 163 mmol/kg dry mass resulted in a 160 % increase in α1AMPK activity and a 145 % increase in α2AMPK activity at rest compared with 909 mmol/kg dry mass in endurance-trained men. However, other investigations have reported no differences in resting AMPK activity in recreationally active men( Reference Steinberg, Watt and McGee 64 , Reference Watt, Steinberg and Chan 65 ), moderately trained men( Reference Roepstorff, Vistisen and Donsmark 66 ) and physically fit men( Reference Camera, West and Burd 29 ), amid modest concentrations of muscle glycogen (approximately 170 mmol/kg dry mass). Furthermore, reduced muscular glycogen concentrations do not appear to hinder post-resistance exercise protein synthesis( Reference Camera, West and Burd 29 , Reference Creer, Gallagher and Slivka 31 , Reference Churchley, Coffey and Pedersen 41 ).
Insulin and protein metabolism
Dietary CHO may also affect protein metabolism by influencing blood insulin levels( Reference Ivy, Ding and Hwang 70 – Reference Fujita, Dreyer and Drummond 72 ). However, although in vitro studies have shown that insulin stimulates muscle protein synthesis via the phosphoinositide 3-kinase (PI3K)-Akt pathway( Reference Trommelen, Groen and Hamer 73 – Reference Bell, Volpi and Fujita 75 ), it appears that insulin holds only a permissive role rather than a modulatory role in enhancing muscle protein synthesis( Reference Abdulla, Smith and Atherton 76 ). In a systemic review and meta-analysis, Abdulla et al.( Reference Abdulla, Smith and Atherton 76 ) found that insulin exerted no stimulatory effect on muscle protein synthesis in the twenty-five studies used. In healthy individuals, the protein synthetic effect of insulin only becomes significant when amino acid delivery is increased; this effect is reduced or nonexistent when amino acid delivery is reduced, even at supraphysiological concentrations of insulin( Reference Abdulla, Smith and Atherton 76 ). Thus, the co-ingestion of CHO with amino acids/protein, as may be practiced by resistance trainees, would provide no additional muscle protein synthetic benefit v. amino acids/protein alone given protein content is adequate (approximately 25 g)( Reference Morton, McGlory and Phillips 77 , Reference Staples, Burd and West 78 ). Staples et al.( Reference Staples, Burd and West 78 ) reported no difference in muscle protein synthesis or eukaryotic initiation factor 4E-binding protein (4E-BP1) and p70S6K phosphorylation at 1 and 3 h post-resistance exercise after ingestion of 25 g of whey protein and 50 g of maltodextrin v. 25 g of whey protein alone 30 min post-exercise. This coincides with data showing that mTORC1 activity in skeletal muscle is unaltered by systemic growth factors that act through the PI3K-pathway, such as insulin and insulin-like growth factor-1, and that post-exercise muscle protein synthesis is an intrinsically mediated process( Reference Watson and Baar 24 , Reference Hornberger, Stuppard and Conley 79 – Reference West, Burd and Staples 81 ).
With regard to muscle protein degradation, however, Abdulla et al.( Reference Abdulla, Smith and Atherton 76 ) concluded that insulin reduces degradation rates and facilitates an overall positive net protein balance. This suppressive effect is mediated by the PI3K-Akt pathway, which is activated by the binding of insulin to its transmembrane insulin receptor on the sarcolemma and initiates translocation of the proteins to the cell membrane( Reference Marcotte, West and Baar 25 ). Akt inhibits forkhead box O, a transcription factor implicated with gene expression associated with the ubiquitin proteasome pathway( Reference Sandri 56 , Reference Latres, Amini and Amini 82 – Reference Mikura, Yamaoka and Doi 85 ) and autophagy( Reference Sandri 56 , Reference van der Vos, Eliasson and Proikas-Cezanne 86 , Reference Meijer, Lorin and Blommaart 87 ), two major pathways of protein degradation, by promoting its exportation from the nucleus into the cytoplasm and inducing its degradation( Reference Coffey and Hawley 50 , Reference Lee, Dai and Hu 84 ). This insulin-mediated reduction in muscle protein breakdown appears to be more potent when amino acids are scarce( Reference Abdulla, Smith and Atherton 76 , Reference Bird, Tarpenning and Marino 88 , Reference Bird, Tarpenning and Marino 89 ). Although Bird et al.( Reference Bird, Tarpenning and Marino 88 , Reference Bird, Tarpenning and Marino 89 ) report a synergistic effect of CHO and amino acid ingestion on attenuating protein degradation, in the presence of adequate amino acid availability, the co-ingestion of CHO with protein following resistance exercise does not appear to result in further suppression of muscle protein breakdown( Reference Morton, McGlory and Phillips 77 , Reference Staples, Burd and West 78 , Reference Greenhaff, Karagounis and Peirce 90 ). Staples et al.( Reference Staples, Burd and West 78 ) showed that except for post-resistance exercise phosphorylation of Akt, after ingestion of 25 g of whey protein and 50 g of maltodextrin, there were no differences in muscle protein degradation or acetyl-CoA carboxylase-β, a surrogate marker for AMPK, v. 25 g of whey protein alone. Reports of enhanced hypertrophy while co-ingesting CHO and protein post-resistance exercise( Reference Candow, Burke and Smith-Palmer 91 – Reference Rahbek, Farup and Moller 93 ) are likely then attributed to amino acid content rather than a combinatory effect of amino acids and CHO and that the resultant insulinogenic response appears to be superfluous in the presence of an adequate provision of amino acids( Reference Morton, McGlory and Phillips 77 , Reference Staples, Burd and West 78 ).
Low carbohydrate intake, muscle glycogen concentrations and cell signalling in response to resistance exercise
The influence of chronic low CHO intakes on post-resistance exercise anabolism has yet to be well characterised. Roberts et al.( Reference Roberts, Holland and Kephart 34 ) conducted a pioneering mechanistic investigation of the effects of a low-CHO ketogenic diet on resistance exercise signalling activity using rats fed either a ketogenic diet (10·3 % CHO, 20·2 % protein, 69·5 % fat) or a Western diet (42·7 % CHO, 15·2 % protein, 42·0 % fat) for 6 weeks. The study showed that a low-CHO ketogenic diet does not impair the acute anabolic responses to resistance exercise. At 90, 180 and 270 min post-exercise, similar rates of muscle protein synthesis and phosphorylation of downstream mTORC1 targets, rps6 and 4E-BP1 in the gastrocnemius were observed in ketogenic-diet-fed rats and Western-diet-fed rats. Phosphorylation of αAMPKThr172 did not increase in either group. There were also no differences in total RNA content, a proxy measure for protein synthetic capacity, or measures of hypertrophy between groups following the 6-week intervention, as both groups incurred significant increases from pre-training levels. It is worth noting, however, that there were no differences in muscle glycogen between groups.
Few data exist pertaining to the cell signalling activity following acute resistance exercise amidst divergent muscular glycogen concentrations. It has been hypothesised that reduced glycogen concentrations augment AMPK activity(
Reference Hawley, Burke and Phillips
1
,
Reference McBride and Hardie
61
) and may blunt mTORC1 activity(
Reference Paoli, Bianco and Grimaldi
67
). In a crossover study by Creer et al.(
Reference Creer, Gallagher and Slivka
31
), eight experienced cyclists underwent 2 consecutive days of glycogen-depleting cycling exercise after having consumed either a very low (2 % of energy intake; 26·0 g/d) or high intake of CHO (80 % of energy intake; 1042·2 g/d) leading to intramuscular glycogen concentrations of 174 and 591 mmol/kg dry mass, respectively. After a 12-h fast, subjects then performed a resistance training bout of three sets of ten repetitions of bilateral knee extension at 70 % of 1RM. Muscle biopsies were taken immediately before exercise, immediately post-exercise and 10 min post-exercise. Pre-exercise phosphorylation levels of mTORC1 targets, AktSer473 (upstream) and p90S6KThr573 (downstream) and mTORC1Ser2448 were not affected by glycogen status at rest. Similarly, phosphorylation levels were not different for any of these proteins between the two glycogen conditions immediately following exercise. In addition, p906SK was unaffected by glycogen status at 10 min post-resistance exercise. However, resistance training in a low-glycogen state resulted in a reduced phosphorylation of Akt compared with completing the same resistance exercise bout with high levels of intramuscular glycogen, but mTORC1 activity was not affected. These findings are partially explained by activity of the load-induced mTORC1 pathways that operate independent of Akt(
Reference Marcotte, West and Baar
25
,
Reference Hornberger, Chu and Mak
94
,
Reference Joy, Gundermann and Lowery
95
). Extracellular signal-regulated kinase (ERK) 1/2
$^{{{\rm Thr185}\,/\,{\rm Tyr187}}} $
, another mediator of cellular growth, was not influenced by glycogen content at rest, immediately post-exercise or 10 min post-exercise. These data suggest that the post-resistance exercise mTORC1 activity is not affected by divergent muscular glycogen concentrations. A study by Churchley et al.(
Reference Churchley, Coffey and Pedersen
41
) investigated the effect of glycogen content on myogenic gene transcription immediately and 3 h post-resistance exercise. Seven strength-trained males underwent a one-legged exhaustive exercise bout to lower muscle glycogen (193 mmol/kg dry mass), whereas the contralateral leg maintained normal glycogen stores (435 mmol/kg dry mass). Participants then completed another bout of resistance training the following day after a 10–12-h fast; each resistance exercise bout consisted of eight sets of five repetitions of leg press (one leg at a time) at 80 % 1RM. There was no significant difference in mRNA abundance of myogenin (MyoG), myogenic differentiation factor D (MyoD) or insulin-like growth factor-1 at rest (before resistance training) between the low and normal leg. Interestingly, mRNA content of atrophy-related genes, atrogin and muscle-specific RING finger (MuRF), was significantly higher in the normal-glycogen leg at rest compared with the low-glycogen leg. In response to resistance training, there were no differences in mRNA abundance of MyoG, MyoD or insulin-like growth factor-1 between legs 3 h post-exercise. There was also no difference in mRNA abundance of atrogin, MuRF or myostatin, demonstrating that glycogen content does not influence transcriptional activity of either hypertrophy- or atrophy-related genes in response to an acute bout of resistance exercise.
Furthermore, Camera et al.( Reference Camera, West and Burd 29 ) also reported similar findings using a near-identical one-legged glycogen-depleting protocol and a resistance training bout of eight sets of five repetitions of leg press (one leg at a time) at 80 % 1RM the following day. Muscle biopsy samples were collected before exercise and 1 and 4 h post-exercise. However, of the sixteen physically fit male subjects used, eight subjects consumed a protein–CHO beverage (20 g of whey protein, 40 g of maltodextrin), whereas eight subjects ingested a placebo (water, artificial sweetener) immediately after exercise and 2 h following completion of the exercise bout. Glycogen content in the reduced glycogen condition was 176 and 184 mmol/kg dry mass, whereas glycogen content in the normal glycogen condition was 382 and 383 mmol/kg dry mass in the placebo and supplement groups, respectively. At rest, no difference in mTORC1Ser2448 phosphorylation was reported between legs or groups, whereas AktSer473 and p70S6KThr389 phosphorylation levels were higher in the low leg compared with the normal leg in both placebo and supplement groups. Resting S6Ser235/236 phosphorylation was significantly greater in the low leg than in the normal leg in the supplement group, but there was no difference in AMPKα Thr172 phosphorylation or atrogin and myostatin mRNA abundance at rest. At both post-exercise time points, no difference in atrogin and myostatin mRNA abundance was seen in the placebo group; however, these same genes were significantly up-regulated in the low-glycogen leg during the post-exercise period in the supplement group. The investigators speculated that rather than preserving muscle protein in the absence of nutrients as in the placebo/fasted condition the provision of exogenous CHO and amino acids in the supplement group amidst the presence of low glycogen may have initiated the muscle remodelling activity. There was no difference in AMPK, Akt, p70S6K and S6 phosphorylation between legs in either group 1 and 4 h post-exercise. mTORC1 phosphorylation was not affected by glycogen status in the placebo group at either post-exercise time point. mTORC1 phosphorylation was significantly increased in both the low-glycogen leg and normal-glycogen leg post-exercise in the supplement group; however, the effect was more pronounced in the normal leg when compared with the glycogen-depleted leg at both 1 h (approximately 4-fold v. approximately 11-fold, respectively) and 4 h post-exercise (approximately 1-fold v. approximately 4-fold, respectively). It is worth noting that activity of downstream targets (p70S6K, S6) was not different between legs, and thus the observed increase in mTORC1 phosphorylation in the low-glycogen leg was sufficient enough to initiate activation of the downstream proteins associated with translation initiation. Further and seemingly most telling, there was no difference in myofibrillar protein synthesis rate between legs in either group during the 1–4 h recovery period (Table 2).
Table 2 A summary of investigations examining the acute post-exercise cellular responses associated with protein synthesis after commencing resistance exercise with divergent levels of muscle glycogen

CHO, carbohydrate; PL, placebo; Pro, protein; AMPK, 5' AMP-activated protein kinase; mTORC1, mammalian target of rapamycin complex 1; MyoG, myogenin; MyoD, myogenic differentiation factor D; IGF-1, insulin-like growth factor-1; MuRF, muscle-specific RING finger; Im, immediately; ERK, extracellular signal-regulated kinase.
These data seem to suggest that low pre-exercise muscle glycogen concentrations (approximately 181·8 mmol/kg dry mass) have negligible effects on the molecular signalling activity of cell growth and protein synthesis in the recovery phase following resistance training. The measured regulatory proteins associated with muscle protein synthesis including mTORC1 and its upstream and downstream targets, as well as rates of protein synthesis, were found to be unaffected by divergent levels of glycogen. The one exception was observed in the investigation by Creer et al.( Reference Creer, Gallagher and Slivka 31 ), which reported a reduced phosphorylation of Akt 10 min post-exercise. However, this may simply be related to the timing of post-exercise biopsies (10 min v. 1, 3 and 4 h)( Reference Camera, West and Burd 29 ). Moreover, this may be of no consequence to resistance-exercise-induced muscle protein synthesis given that mechanical-stimulated mTORC1 activity occurs independent of the Akt pathway( Reference Watson and Baar 24 , Reference West, Burd and Staples 81 ). Therefore, it appears that the resistance-exercise-induced activation of anabolic cell signalling is potent enough to overcome any potential inhibitory signalling activity elicited by low glycogen concentrations, should any exist. This is consistent with the observation that the repressive effects of AMPK on anabolic signalling activity and muscle protein synthesis in humans appears to be negligible following exercise, especially in the later stages of recovery (i.e. 1–4 h)( Reference Camera, West and Burd 29 , Reference Dreyer, Fujita and Cadenas 57 , Reference Lundberg, Fernandez-Gonzalo and Tesch 59 , Reference Wilkinson, Phillips and Atherton 60 , Reference Apro, Moberg and Hamilton 96 , Reference Coffey, Zhong and Shield 97 ).
Amino acids, resistance exercise and protein balance
Protein feeding acutely increases the free amino acid pool and exerts a profound effect on muscle protein balance by increasing protein synthesis and reducing protein degradation( Reference Areta, Burke and Ross 98 – Reference Tipton, Gurkin and Matin 102 ). A provision of essential amino acids (6–12 g), particularly leucine (3–6 g), favourably influences muscle protein synthesis over a period of hours( Reference Morton, McGlory and Phillips 77 , Reference Tipton, Borsheim and Wolf 100 , Reference Burd, West and Moore 101 , Reference Phillips, Tang and Moore 103 ), by not only serving as substrates to be incorporated into new proteins but by up-regulating mTORC1 activity as well( Reference Kimball and Jefferson 104 – Reference Meijer, Lorin and Blommaart 106 ). When intracellular amino acid content increases, amino acids accumulate within the lysosome, initiating the translocation of mTORC1 to the membrane of the lysosome where it interacts with its activator Ras homologue enriched in bran (Rheb)( Reference Marcotte, West and Baar 25 , Reference Bar-Peled and Sabatini 107 , Reference Efeyan, Zoncu and Chang 108 ). Although it has yet to be fully characterised, amino-acid-stimulated movement of mTORC1 to the lysosome is mediated by the Rag family of G-proteins (RagA, B, C, D) where the presence of amino acids results in the activation of this complex, which binds to raptor, the regulatory protein of mTORC1, and recruits mTORC1 to the lysosome through its interaction with the Ragulator on the lysosomal membrane( Reference Watson and Baar 24 , Reference Sancak, Bar-Peled and Zoncu 109 ). Amino acids can also inhibit autophagy( Reference Deldicque, Theisen and Francaux 105 , Reference Meijer, Lorin and Blommaart 106 , Reference Kanazawa, Taneike and Akaishi 110 , Reference Sugawara, Ito and Nishizawa 111 ) and the ubiquitin proteasome pathway( Reference Herningtyas, Okimura and Handayaningsih 112 , Reference Sadiq, Hazlerigg and Lomax 113 ), two intracellular pathways implicated with the degradation of cellular proteins. Even modest amounts of intact proteins (approximately 20–25 g) rich in essential amino and/or branched-chain amino acids are capable of optimally stimulating muscle protein synthesis( Reference Morton, McGlory and Phillips 77 , Reference Moore, Robinson and Fry 114 , Reference Yang, Churchward-Venne and Burd 115 ). Indeed, it has been observed that a 20-g dose of protein every 3 h over a 12-h period substantially increases synthesis rates of protein in skeletal muscle and promotes overall positive balance of protein in the body( Reference Areta, Burke and Ross 98 , Reference Moore, Areta and Coffey 116 ). This effect seems to be unaltered by CHO intake. Harber et al.( Reference Harber, Schenk and Barkan 117 ) showed that 7 d of a very-low-CHO-higher-protein diet (5 % CHO, 60 % fat and 35 % protein) increased muscle fractional synthetic rate 2-fold compared with an isoenergetic (117 kJ/kg body mass (28 kcal/kg body mass)) traditional Western diet (60 % CHO, 30 % fat, 10 % protein) in healthy subjects. Furthermore, a study by Robinson et al.( Reference Robinson, Jaccard and Persaud 118 ) measured whole-body protein turnover for 9 h in seven men who were fed hourly isoenergetic beverages providing 70 % of total energy from CHO or protein. All seven men completed both trials (in addition to a fasted trial). Protein synthesis in response to the high-CHO beverage was 132·2 g, whereas degradation was 109·8 g, resulting in a net nitrogen balance of +21·4 g over the 9 h. However, protein synthesis in response to the high-protein beverage was 243·9 g, and a degradation of 78·9 g, resulting in a net nitrogen balance of +165 g. Corresponding CHO intake during trials was 291 and 62 g for the high-CHO trial and high-protein trials, respectively. These data in addition to the documented modest effect of CHO ingestion on protein degradation( Reference Abdulla, Smith and Atherton 76 , Reference Bird, Tarpenning and Marino 88 , Reference Bird, Tarpenning and Marino 89 ) demonstrate that sufficient protein intake can result in a positive muscle protein balance, which is necessary for hypertrophy, and is not predicated on CHO ingestion.
Finally, as mentioned earlier, resistance exercise significantly affects muscle protein metabolism. Resistance training elicits an intrinsic stimulatory response to mTORC1, independent of the PI3K-Akt pathway, by activating a currently unknown kinase that phosphorylates tuberous sclerosis complex 2 (TSC2) within an RxRxxS*/T* motif and initiates its translocation away from the lysosome allowing mTORC1 to interact with Rheb( Reference Watson and Baar 24 , Reference Marcotte, West and Baar 25 ). In addition, phosphatidic acid, a glycerophospholipid, also mediates load-induced mTORC1 activation, potentially through direct binding to the 12-kDa FK506-binding protein (FKBP12)-rapamycin-binding domain of mTORC1; however, the origin of synthesis of phosphatidic acid in response to mechanical loading also has yet to be identified( Reference Watson and Baar 24 , Reference Marcotte, West and Baar 25 , Reference Joy, Gundermann and Lowery 95 ). Resistance exercise results in elevated rates of protein synthesis immediately post-exercise and up to 24–48 h following an acute bout( Reference Dreyer, Fujita and Cadenas 57 , Reference Biolo, Maggi and Williams 119 – Reference Moore, Tang and Burd 122 ). Furthermore, it has been well established that this response can be enhanced by protein ingestion in the proximal hours before and after exercise( Reference Aragon and Schoenfeld 47 , Reference Churchward-Venne, Murphy and Longland 99 , Reference Biolo, Maggi and Williams 119 , Reference MacDougall, Gibala and Tarnopolsky 123 – Reference Phillips 126 ) in addition to decreasing the protein degradation observed following resistance exercise when performed in the fasted state( Reference Tipton, Ferrando and Phillips 127 ). Given that skeletal muscle mass is primarily dictated by the regulation of protein synthesis( Reference Marcotte, West and Baar 25 , Reference Greenhaff, Karagounis and Peirce 90 ), the synergistic effect of timely and adequate protein intake in combination with resistance training appears to be sufficient enough to promote activation of the requisite molecular machinery for facilitating skeletal muscle hypertrophy( Reference Watson and Baar 24 , Reference Marcotte, West and Baar 25 ) irrespective of CHO intake and/or glycogen status( Reference Camera, West and Burd 29 , Reference Creer, Gallagher and Slivka 31 , Reference Churchley, Coffey and Pedersen 41 ).
Discussion
Despite the traditional view that regularly training individuals should consume a CHO-rich diet( Reference Kreider, Wilborn and Taylor 27 , Reference Kraemer, Adams and Cafarelli 128 ), need should be determined by the influence of CHO on the acute energetic support of exercise, as well as the implications on the cellular signalling activity that underlie chronic adaptation. Of which, resistance exercise is metabolically distinct from endurance exercise and the expression of adaption-based genes is mediated through divergent pathways, thus CHO need may also be distinct. It has been suggested that individuals who engage in regular resistance training should consume a moderate to high daily intake of CHO (i.e. 3–7 g/kg per d)( Reference Slater and Phillips 14 , Reference Lambert, Frank and Evans 28 ); however, recent investigation suggest that intakes of CHO lower than what is commonly recommended may still allow for optimal resistance exercise adaptations( Reference Helms, Aragon and Fitschen 13 ). As such, the current paradigm of CHO requirements with regular resistance training appears to be inconsistent with experimental observations yielded from such studies.
First, it has not been observed that regular resistance-based exercise bears the potential to lead to true glycogen depletion. And, given that glycogen replenishment may occur even in an unfed state( Reference Robergs, Pearson and Costill 36 , Reference Pascoe and Gladden 46 ), it is likely that even modest daily intakes of CHO are capable of restoring glycogen stores. In addition, it has been demonstrated that during periods of low CHO intake and/or low muscle glycogen concentrations, resistance exercise performance is maintained (i.e. 1RM, training volume)( Reference Mitchell, DiLauro and Pizza 30 , Reference Sawyer, Wood and Davidson 32 ), whereas early data may also suggest unhindered hypertrophy( Reference Roberts, Holland and Kephart 34 ). Furthermore, anabolic cell signalling( Reference Camera, West and Burd 29 , Reference Creer, Gallagher and Slivka 31 ), gene transcription( Reference Churchley, Coffey and Pedersen 41 ) and muscle protein synthesis( Reference Camera, West and Burd 29 ) also appear unimpaired. The investigations of intracellular signalling activity corresponding to glycogen content and resistance training suggest that resistance exercise- and amino-acid-mediated protein synthetic responses are unaffected by low levels of muscle glycogen( Reference Camera, West and Burd 29 , Reference Creer, Gallagher and Slivka 31 , Reference Churchley, Coffey and Pedersen 41 ). Given that adaptation to training results from the cumulative effect of transient increases in mRNA transcripts that encode for proteins after each successive exercise bout( Reference Hawley, Hargreaves and Joyner 23 ), the unimpaired resistance exercise and/or amino acid feeding-induced up-regulation of regulatory proteins (mTORC1, p70S6K, 4E-BP1) associated with gene translation, gene transcription and protein synthesis may allow for uncompromised adaptation. Further, insulin appears to play only a permissive role in muscle protein synthesis rather than an augmentative one( Reference Abdulla, Smith and Atherton 76 ). And, while insulin may reduce protein degradation( Reference Abdulla, Smith and Atherton 76 ), an adequacy of amino acids may accomplish the same effect while concomitantly promoting protein synthesis( Reference Abdulla, Smith and Atherton 76 – Reference Staples, Burd and West 78 ). Thus, it appears that the insulinogenic response elicited by CHO ingestion is not required to activate or enhance the protein synthetic response in the presence of sufficient amino acids( Reference Abdulla, Smith and Atherton 76 – Reference Staples, Burd and West 78 ).
Further inquiry is needed to establish the role of CHO intake in resistance-based exercise adaptation including hypertrophy and strength, as limited research is currently available. Moreover, many discrepancies lie between the works that currently exist, particularly with regard to nutritional intervention implementation. Not all studies used isoenergetic diets, nor were all investigations matched for protein intake. Additionally, many of the studies did not provide meals to subjects during interventions and relied upon self-reported diet adherence. Further, the relatively small samples used in these studies may have prohibited small, but meaningful, effects from being detected between CHO and glycogen conditions. Because of these limitations, it is premature at the present time to amend CHO recommendations, nonetheless these data do call into question the current view of CHO need as both a substrate for resistance training and a modulator of the cellular signalling activity that underlie resistance training adaptation.
Acknowledgements
This research received no specific grant from any funding agency, commercial or not-for-profit sectors.
K. A. E., T. A. V., C. M. K. developed the topic for review; K. A. E. and T. A. V. conducted the review and drafted the paper; C. M. K. provided direction and feedback during drafting of the paper, including edits of various drafts of the article.
The authors declare that there are no conflicts of interest.