Introduction
The most important cause of mortality in great apes in zoological settings is cardiovascular disease (CVD), affecting all four taxa: chimpanzee (Pan troglodytes), bonobo (Pan paniscus), gorilla (Gorilla spp.) and orangutan (Pongo spp.)(Reference Murphy, Danforth and Clyde1,Reference Murphy and Danforth2) . Mortality reviews from zoological institutions have reported that CVD is the cause of death in 31–77% of chimpanzees, 65% of bonobos, 27–41% of gorillas and 16–29% of orangutans. Great ape CVD predominately, but not exclusively, affects male individuals in the adult or geriatric age groups(Reference Murphy, Danforth and Clyde1–Reference Laurence, Kumar and Owston4).
The most typical histological characterisation of CVD in great apes is myocardial fibrosis in the absence of coronary infarction(Reference McManamon and Lowenstine5). A certain amount of myocardial fibrosis, as part of cardiovascular degenerative processes, can be expected with ageing and can be considered as normal(Reference Susic and Frohlich6). However, histological examinations have revealed that cardiac changes, reflected by myocardial fibrosis, are typically more advanced than can be attributed to normal ageing processes(Reference McManamon and Lowenstine5,Reference Lowenstine, McManamon and Terio7) . In addition, CVD has also been observed in non-geriatric great apes, especially in male chimpanzees(Reference Murphy, Danforth and Clyde1,Reference Strong, Martin and Redrobe3,Reference Seiler, Dick and Guardado-Mendoza8) . Left ventricular hypertrophy and atrial dilation are common macroscopic findings in great ape CVD, whereby high grades of myocardial fibrosis may result in progression to cardiac enlargement and dilation, consistent with chronic congestive heart failure(Reference Murphy, Danforth and Clyde1,Reference Murphy and Danforth2,Reference Lammey, Lee and Ely9) .
Clinical signs of great ape CVD are usually subtle, including lethargy, anorexia, weight changes, avoidance of antagonistic or aggressive interactions with conspecifics and loss of social ranking. When the disease progresses, weight gain, peripheral oedema and respiratory distress may develop as signs of decompensated congestive heart failure, but more frequently sudden death due to arrhythmias is noticed(Reference Murphy, Danforth and Clyde1–Reference Strong, Martin and Redrobe3,Reference Seiler, Dick and Guardado-Mendoza8,Reference Lammey, Lee and Ely9) .
As a result of the poor clinical conditions and the high mortality rates, understanding of the aetiopathogenesis of great ape CVD has been an important topic. Multiple studies have recently discussed a range of potential causative factors that may contribute to myocardial fibrosis. The majority of these authors stated that the disease is most likely multifactorial in origin. The highlighted factors such as hypertension, obesity, vitamin and mineral imbalances, impaired endocrine signalling, chronic stress (e.g. due restricted spatial environment, executing unnatural behaviour…) and concomitant diseases, such as renal disease, all play a role in a complex model, potentially underlying the development of myocardial fibrosis and subsequent deterioration of the cardiac function(Reference Strong, Martin and Redrobe3–Reference McManamon and Lowenstine5,Reference Lowenstine, McManamon and Terio7,Reference Seiler, Dick and Guardado-Mendoza8) .
Many, if not all, causative factors are directly or indirectly related to the diet which in wild-living great apes consists mainly of fibre-rich, low-carbohydrate and very low-sodium components(Reference Conklin-Brittain, Knott and Wrangham10–Reference Schmidt and Shaw12). Compared with the situation in the wild, diets of great apes housed in zoological settings are often different with various consequences for the cardiovascular function. Moreover, vitamin D deficiency has recently been recognised to be of frequent occurrence in great apes housed in more northern regions and current dietary/supplementary guidelines, to maintain a correct vitamin D status are under discussion(Reference Videan, Heward and Fritz13–Reference Moittié, Jarvis and Bandelow15). Although additional research is needed, recent hypotheses indicate that vitamin D deficiency is a plausible underlying factor in the pathogenesis of myocardial fibrosis in great apes.
This literature overview: (i) examines important differences in nutritional factors between zoological and wild great ape populations; (ii) explains the detrimental effects of the highlighted dietary discrepancies on cardiovascular function in great apes; and (iii) elucidates specific nutrition-related pathophysiological mechanisms that may underlie the development of myocardial fibrosis. This information can contribute to the understanding of the aetiopathogenesis of myocardial fibrosis in great apes and pave the way for future clinical studies and a more preventive approach to CVD management in great apes.
Cardiovascular disease prevalence in free-roaming great apes
Comparison of prevalence figures and potential contributing factors that differ between populations under human care and animals of the same species living in their natural habitats can be of great value. Unfortunately, pathological information about free-ranging great apes is limited, but one study, involving chimpanzees (median age 20 years) from Gombe National Park in Tanzania, showed that myocardial fibrosis is less common (2/11)(Reference Terio, Kinsel and Raphael16). Specifically, the identified lesions indicated fibrosis originating from intramyocardial arterioles and extending into the myocardial tissue, aligning with those observed in animals under human care, albeit milder in severity(Reference Terio, Kinsel and Raphael16). Yet surprisingly, cardiac samples from African sanctuary chimpanzees (n = 25) showed no evidence of myocardial fibrosis with the remark that investigated animals were relatively young (median 12 years)(Reference Strong, Moittié and Sheppard17). However, myocardial fibrosis has already been observed in young and sub-adult animals in European zoos, suggesting that age alone cannot explain the difference between the two populations(Reference Strong, Martin and Redrobe3). Post-mortem examinations in wild gorillas showed inconsistent findings: only 3% of mountain gorillas (Gorilla beringei beringei) showed histological changes specific to myocardial fibrosis, which is in contrast to the high incidence found in 75% (6/8) of wild eastern lowland gorillas (Gorilla beringei graueri) in the Democratic Republic of the Congo(Reference Lowenstine, McManamon and Terio7,Reference Kambale, Ramer and Gilardi18) .
Myocardial fibrosis: the hallmark of cardiovascular disease in great apes
Keeping in mind that humans are the closest evolutionary relatives of great apes, literature on related identities of cardiovascular disease in humans can be analysed in the search for relevant extrapolations. Despite this line of thought, only limited or focal myocardial fibrosis is observed in human patients with no recognised equivalent of the condition seen in great apes. Remarkably, the most common cardiovascular disease in humans, coronary artery atherosclerosis is observed solely with low frequency and mild intensity in great apes and therefore never leading to myocardial infarction as a cause of death(Reference Díez19,Reference Varki, Anderson and Herndon20) . Noteworthily, varying degrees of median hypertrophy and sclerosis were observed in endomyocardial (intrinsic) coronary arteries and arterioles of affected great apes(Reference Lowenstine, McManamon and Terio7).
Myocardial fibrosis is a common outcome due to non-specific responses to cardiac insults. Different histological types are generally being described in affected hearts of great apes, including interstitial fibrosis, perivascular fibrosis and replacement fibrosis(Reference Strong, Martin and Redrobe3,Reference Lowenstine, McManamon and Terio7,Reference Strong, Moittié and Sheppard17) . Interstitial and perivascular fibrosis are characterised by an increased accumulation of extracellular matrix (ECM) (e.g. collagen I and III proteins), produced by fibroblasts mostly without significant cardiomyocyte damage or death(Reference Doppler, Carvalho and Lahm21,Reference Hinderer and Schenke-Layland22) . Formation of interstitial and perivascular cardiac fibrosis is considered as a reactive response to underlying conditions such as hypertension, diabetes mellitus, inflammation, renal disease and ageing-related processes(Reference Susic and Frohlich6,Reference Cuspidi, Ciulla and Zanchetti23,Reference Hulshoff, Rath and Xu24) . Conversely, replacement fibrosis is a direct result of cardiomyocyte damage and can generally be caused by myocarditis or myocardial infarction, but in great apes it is most likely a consequence of excessive interstitial fibrosis and therefore used as a marker of disease progression(Reference Terio, Kinsel and Raphael16,Reference Strong, Moittié and Sheppard17,Reference Frangogiannis25) . One of the most common and best-known causes appointed to similar types of cardiac fibrosis in humans is systemic hypertension(Reference Díez19,Reference Frangogiannis25,Reference Jellis, Martin and Narula26) . The echocardiographic presence of concentric left ventricular hypertrophy, as well as intrinsic coronary artery hypertrophy, is also substantial evidence to suggest hypertension as an important underlying factor for CVD in great apes(Reference Murphy and Danforth2,Reference Lowenstine, McManamon and Terio7,Reference Strong, Moittié and Sheppard17) .
Ely and colleagues (2011) showed in a population of 231 chimpanzees that, after 7 years of follow-up, animals with elevated systolic blood pressures had a relative risk of all-cause mortality of 2·60, compared with normotensive animals(Reference Ely, Zavaskis and Lammey27). However, data on the relative mortality risk specific to CVD or associations between systolic blood pressure and the degree of myocardial fibrosis have not been published to date. The authors estimated systolic blood pressure reference intervals in this healthy group of chimpanzees and defined that normal systolic blood pressure ranged up to 147 mmHg, with a prehypertensive range of 148–153 mmHg (90th percentile), and values above 154 mmHg were considered hypertensive (95th percentile). Remarkably, these reference intervals are substantially higher compared with those in humans (prehypertensive 120–139 mmHg; hypertension >140 mmHg)(Reference Pickering, Hall and Appel28). Moreover, a significant increase in the risk of all-cause mortality was already observed in the prehypertensive range, highlighting a possible overestimation of the actual reference values(Reference Ely, Zavaskis and Lammey27).
Dietary selection in free-roaming great apes
Important factors contributing to myocardial fibrosis, such as hypertension, obesity and the metabolic syndrome, are strongly related to the diet(Reference Murphy, Danforth and Clyde1,Reference Yancy, Jessup and Bozkurt29) . Therefore, it is useful to get a better view on what foods these species naturally eat in the wild. Most knowledge regarding adequate nutritional needs is derived from observations of dietary habits of free-ranging animals and by comparing the anatomy of the gastrointestinal tract of the different great ape species(Reference Schmidt and Shaw12,Reference Stevens and Hume30) . Diet composition of free-ranging great apes consists mainly of plant-based matter, with clear differences between great ape species.
Chimpanzees and bonobos, both members of the genus Pan, are classified as omnivorous frugivores and consume similar plant-based food sources consisting mainly of high-fibrous fruits, although big parts of the diet also include leaves, flowers, seeds, shoots and stems(Reference Conklin-Brittain, Knott and Wrangham10,Reference Gerstner and Pruetz31) . These species also share a very similar gastrointestinal anatomy with a relatively short small intestine and a more pronounced large intestine, suggesting that fibre fermentation, as part of the energy source, cannot be neglected(Reference Schmidt and Shaw12,Reference Nishida and Uehara32) . It is worth noting that chimpanzees and bonobos also tend to eat meat and insects, since digested substances of animal origin are often found in faecal sample analysis; moreover, active hunting behaviour is reported(Reference McGrew33). Although both species show a carnivorous tendency, the protein contribution of meat in the diet is too low to be considered significant, which is not the case for insect matter(Reference Deblauwe and Janssens34). Indeed, O’Malley and Power showed that various forms of insectivory offered small, yet likely meaningful, amounts of micro- and macronutrients (i.e. fat and protein)(Reference O’Malley and Power35,Reference O’Malley and Power36) .
Gorillas’ and orangutans’ gastrointestinal tracts are characterised by a more capacious colon, in comparison with great ape species from the genus Pan, which emphasises their ability to digest fibre-rich foods. Fermentative capabilities of all great apes are particularly important during the dry season when fibrous foods of lower nutritional quality are consumed(Reference Schmidt and Shaw12,Reference Stevens and Hume30,Reference Williamson, Tutin, Rogers and Fernandez37) . The most herbivorous of all great apes are gorillas. Especially mountain gorillas (Gorilla beringei beringei) consume almost exclusively browse including leaves, stems, pith and shoots, but the diet composition clearly differs between different gorilla species(Reference Ganas, Robbins and Nkurunungi38). Other gorilla species, such as eastern lowland gorillas (Gorilla beringei graueri), and especially western lowland gorillas (Gorilla gorilla gorilla), tend to have more diverse diets depending on the seasonal variety, whereby fruits can make up to significant amounts of their diets(Reference Yamagiwa, Mwanza, Yumoto and Maruhashi39,Reference Rogers, Abernethy and Bermejo40) . Moreover, insectivory is also observed in western lowland gorillas; however, the average prey biomass intake per day by chimpanzees is considered twice that by gorillas(Reference Deblauwe and Janssens34–Reference O’Malley and Power36). Orangutan species have similar seasonal feeding habits to the latter gorilla species with a preference for fruits when available. Nevertheless, vegetation such as leaves, inner bark, flowers and other plant-based material counts as an important part of their diets(Reference Schmidt and Shaw12,Reference Galdikas41,Reference Vogel, Alavi and Utami-Atmoko42) .
Dietary sodium in the natural diet
One of the current and most recommended dietary strategies to lower blood pressure in humans is reducing sodium intake(Reference Appel, Brands and Daniels43). Sodium sources in tropical habitats are scarce because tropical soils and most plant parts contain low levels of sodium(Reference Van Wambeke44,Reference Rothman, Van Soest and Pell45) . This implicates that diets consumed by great apes in natural environments are low in sodium. Current understanding about natural sodium intake in great apes is derived mainly from investigations in mountain gorillas (Gorilla beringei beringei). For example, the group of Grueter (2013) showed that the most eaten plants by mountain gorillas in Volcanoes National Park, Rwanda contain relatively little sodium (<70 mg/kg on dry matter basis)(Reference Grueter, Ndamiyabo and Plumptre46). Mean sodium concentrations in plant parts and fruits, eaten by mountain gorillas in Bwindi Impenetrable National Park, Uganda, were determined to be 90·4 mg/kg on a dry matter basis(Reference Rothman, Van Soest and Pell45). In another study by Rothman et al. (2007) concerning mountain gorillas in Bwindi Impenetrable National Park, Uganda, daily sodium intake was estimated and the mean values for adult males and females (mg/kg0·75) were 0·05 and 0·06, respectively(Reference Rothman, Plumptre and Dierenfeld11). This signifies a mean daily sodium uptake of 2·75 mg in males and 1·98 mg in females (considering a mean body weight of 195 kg and 100 kg, respectively). A certain risk of sodium deficiency in gorillas, and probably other great apes, would be presumed on the basis of previous data.
Remarkably, different strategies that ensure adequate sodium acquisition were observed in most great ape species. Mountain gorillas have been observed feeding on decaying wood which contains much higher sodium values, with a mean value of 810 mg/kg on a dry matter basis. Wood consumption has been observed on 35 of 319 d, at least 1 d per month with an estimated percentage of 3·9% of total wet weight uptake. Surprisingly, this behaviour contributes to an average of 95·6% of total dietary sodium intake(Reference Rothman, Van Soest and Pell45). Other researchers reported a similar behaviour whereby mountain gorilla groups were sighted, foraging on community lands to feed on barks of eucalyptus, which contains 3100 mg Na/kg on a dry matter basis. One group obtained 73% of their total sodium intake out of eucalyptus barks, even though they only stayed there for a limited time(Reference Grueter, Ndamiyabo and Plumptre46). Chimpanzees in Budongo, Uganda, ate pith of decaying palm trees as a source of sodium(Reference Reynolds, Lloyd and Babweteera47). Harvest of such trees forced the animals to search for another sodium source, whereby eventually eucalyptus bark was consumed, a similar behaviour pattern as seen in gorillas(Reference McCarthy, Lester and Stanford48). Consuming sodium-rich plants growing at great heights, such as Lobelia spp., is another known strategy of mountain gorillas to acquire a sufficient sodium uptake(Reference Grueter, Ndamiyabo and Plumptre46). It is supposed that Bornean orangutans (Pongo pygmaeus) rely on geophagy to obtain sodium, as they have been observed eating soil or using natural licks(Reference Matsubayashi, Ahmad and Wakamatsu49). Moreover, seasonal insectivory, such as termite fishing, offers chimpanzees the opportunity to address diverse mineral requirements. Nevertheless, when compared with other minerals, the sodium levels in preyed insects are relatively low, ranging from 100 to 900 mg/kg (dry matter basis), resulting in an average daily sodium intake estimated to be between 0·8 and 13·8 mg, depending on the specific insect species(Reference O’Malley and Power36). These observations show a common behaviour in great apes, namely searching for items rich in sodium at regular intervals, both in rainy and in dry seasons. It is suggested that these animals look for sodium sources once they tend to become deficient, although additional studies are required to confirm these observations. Nevertheless, sodium values in diets of great apes may be higher than previously assumed with a sodium intake in mountain gorillas on wood consumption days of 73 mg in males and 64 mg in females (considering a mean body weight of 195 kg and 100 kg, respectively)(Reference Rothman, Plumptre and Dierenfeld11).
The National Research Council (NRC) recommends diets with a sodium value of 0·2% (or 2000 mg/kg), on a dry matter basis, to support the maintenance of primates with the remark that these values are likely to exceed minimum needs(50). Remarkably, apart from specific foods like eucalyptus bark, the sodium content of natural diets is extremely low in comparison with the suggested requirements, indicating that great apes are adapted to periods of very low dietary sodium concentrations(Reference Cancelliere, DeAngelis and Nkurunungi51). If the estimated values of daily sodium uptake in free-ranging great apes are correct, a situation of enduring sodium excess in great apes in zoological settings can be assumed as often large quantities of primate maintenance pellet were or are still provided. Until recently, a typical ‘as fed’ feeding programme consisted of 75% produce/browse and 25% primate maintenance pellet, while most primates daily consume 2–4% of their body weight on a dry matter basis(Reference Schmidt and Shaw12,52) . The nutrient composition of a typical primate pellet consists of an average of 0·3% sodium, on a dry matter basis, and a maximum of 12% moisture, meaning that, for example, an estimated daily consumed quantity of 1000 g pellets for a 70 kg chimpanzee contains approximately 2640 mg sodium(50,52) . Therefore, a large excess of dietary sodium can be expected compared with the natural diet, potentially dysregulating the homeostatic balances.
Sodium and the effect on the systolic blood pressure in great apes
A chronic excessive sodium intake in humans is associated with increased systolic blood pressure, cardiovascular events and premature death(Reference Appel, Brands and Daniels43,Reference Sacks, Svetkey and Vollmer53) . To the best of our knowledge, there have been no specific studies conducted looking for associations between dietary sodium intake and the development of CVD in great apes. However, several studies investigating the effect of dietary sodium on the systolic blood pressure have been published in chimpanzees. Elliot and colleagues (2007) conducted dietary experiments in two different groups of chimpanzees(Reference Elliott, Walker and Little54). Interestingly, already at baseline an appreciable difference in systolic blood pressure between these two groups was observed. Based on the human hypertension cut-off of 140 mmHg, only 11·8% of the animals of the first group (n = 17) were considered hypertensive whereas in 42·7% of the animals of the second group (n = 110) hypertension was diagnosed. The baseline level of daily sodium intake in the first group (1718 mg) differed notably compared with the second group (5679 mg). This difference in dietary sodium intake might at least partially explain the discrepancy between the percentage of hypertensive animals between the two groups at baseline(Reference Elliott, Walker and Little54). Indeed, when dietary sodium was reduced to 2885 mg in fifty chimpanzees of the second group, a decrease of 10·9 mmHg in systolic blood pressure was observed after a 2-year period compared with the control group (n = 60)(Reference Elliott, Walker and Little54).
In the first group of animals in the study by Elliot et al., hypertension was diagnosed in only a relatively small percentage of animals (according to the human reference intervals), indicating appropriate dietary sodium levels. However, a longitudinal reduction in daily sodium intake from 1718 mg to 805 mg was still able to significantly lower systolic blood pressure by 5·3 mmHg in the first group of animals(Reference Elliott, Walker and Little54). Comparable findings were observed in a study conducted by Denton et al. (1995) in twenty-six chimpanzees, investigating the consequences of increasing sodium supplementation(Reference Denton, Weisinger and Mundy55). During the first weeks of the study, 5 g of salt or 1995 mg of sodium (as the single variable) was added to the diet of ten animals (treatment group). The diet was previously very low in sodium in accordance with the situation in the wild and consisted only of fruits and vegetables. Interestingly, after 19 weeks, mean systolic pressure increased by 12 mmHg and the body weight increased by 3·6 kg compared with the control group (n = 16). Over time, additional sodium was added to the diet of the treatment group, leading to a progressive increase in blood pressure in most animals. The highest sodium intake (5911 mg) severely elevated the mean systolic pressure by 33 mmHg. Twenty weeks after discontinuation of added sodium, the blood pressure of the treatment group had fallen to baseline and control values(Reference Denton, Weisinger and Mundy55).
Therefore, the authors concluded that chimpanzees should be fed a diet containing no more than 700–900 mg of sodium per day(Reference Denton, Weisinger and Mundy55). Updated dietary guidelines for great apes in zoological institutions were only recently published. The authors recommend providing only 1115% of a specific primate maintenance pellet on an ‘as fed’ basis(Reference Schmidt and Shaw12,Reference Cox, Carlsen, de Jongh and Pluháčková56) . The daily intake of sodium in such feeding programmes is in line with the nutritional recommendations of Denton et al., but a sodium surplus compared with the situation in the wild can still be assumed. Whether this degree of chronic extra sodium intake has long-term negative health effects in great apes is still unclear.
Salt sensitivity, renal function, stress and the repercussions on the cardiovascular function
Despite the body’s ability to handle transient sodium fluctuations, degenerative changes begin to develop when a salt excess persists(Reference Appel, Brands and Daniels43). The study of Denton and colleagues revealed that not all chimpanzees showed a significant rise in blood pressure during the period of extra salt addition(Reference Denton, Weisinger and Mundy55). This variable response to salt loading is well known in human medicine, under the title of ‘salt-sensitivity’ or ‘salt-sensitive hypertension’ whereby salt-sensitive hypertensive patients are three times more likely to develop cardiovascular diseases(Reference Guyton, Coleman and Cowley57,Reference Morimoto, Uzu and Fujii58) . A pivotal mechanism, behind the chronic state of salt-sensitive hypertension, is characterised by a decrease in renal excretory function or an increase in renal sodium reabsorption and, thus, disruption of the maintenance of the sodium and water balance(Reference Guyton, Coleman and Cowley57,Reference Morimoto, Uzu and Fujii58) . In this pathophysiological context of persistent renal sodium and fluid retention tendency, multiple underlying factors may play a role, whereby the associations with oxidative stress, intrarenal inflammation/fibrosis and angiotensin activity are considered most important(Reference Rodriguez-Iturbe, Romero and Johnson59,Reference Majid, Prieto and Gabriel Navar60) .
In humans, the increased risk of hypertension and left ventricular hypertrophy with concomitant renal dysfunction (further defined as chronic kidney disease (CKD)) has been well documented. Even patients with mild impairment of renal function are more prone to CVD and death, and with advancement of the CKD stage there is a corresponding increase in risk of heart failure(Reference Hillege, Nitsch and Pfeffer61,Reference Hill, Fatoba and Oke62) . Renal fibrosis in great apes is characterised by similar histological changes as in cardiac fibrosis. Increased ECM deposition with glomerulosclerosis (GS), tubulointerstitial fibrosis and loss of renal corpuscles and tubules are the hallmarks of CKD in great apes(Reference Chilton, Wilcox and Lammey63). In a mortality review of captive orangutans (n = 122) cardiovascular disease, respiratory infections and renal disease were the most common causes of death. The only association was found between cardiovascular and renal disease(Reference Lowenstine, McManamon and Terio7). Post-mortem examinations performed in ninety-one chimpanzees also revealed a significant association between cardiac and renal fibrosis, as both pathologies were observed simultaneously in fifty-eight of ninety-one animals (63%). The authors concluded that animals with minimal to mild glomerulosclerosis had increased risk of dying from heart failure versus animals without glomerulosclerosis(Reference Chilton, Wilcox and Lammey63). Conversely, hypertension can also be an underlying cause of CKD; if one system’s function starts to deteriorate, the other is affected by its consequences, which in turn further contribute to the dysfunction of the first(Reference Hulshoff, Rath and Xu24).
Chronic stress is a recognised consequence of keeping great apes under human care, and its impact in the development of salt-sensitive or other types of hypertension should not be ignored(Reference Clark64). In humans, for example, salt-sensitive individuals respond with a higher increase in heart rate to mental stress compared with the control group(Reference Buchholz, Schächinger and Wagner65). Moreover, salt-sensitive individuals tend to exhibit elevated urinary epinephrine levels, indicating that a high sympathetic state may be at least partially responsible for the salt-sensitive phenotype(Reference Miyajima and Yamada66). Indeed, Dibona (2004) stated that increased renal sympathetic nerve activity (RSNA) is known to be a factor capable of decreasing renal excretory function(Reference Dibona67). One of the consequences of increased RSNA is an increased renal tubular sodium reabsorption leading to fluid retention(Reference Lalioti, Zhang and Volkman68,Reference Mu, Shimosawa and Ogura69) . Renal vasoconstriction is another direct consequence of an increased RSNA, leading to a decreased renal blood flow, which results in a decline of the pressure dependent natriuresis. Normally, as seen in salt-resistant individuals, high sodium intakes lead to a physiological renin–angiotensin–aldosterone (RAS) system suppression. However, RSNA leads to an increased renin release, provoking the RAS system and eventually mediating Ang II production. Ang II contributes to salt-dependent hypertension by enhancement of sodium resorption in the proximal convoluted tubule(Reference Brooks, Haywood and Johnson70–Reference Guild, McBryde and Malpas72). Obesity is also associated with increased sympathetic nervous activity and RSNA, indicated by elevated renal norepinephrine values(Reference Rumantir, Vaz and Jennings73,Reference Grassi, Seravalle and Dell’Oro74) . Due to low fibre and high simple carbohydrate intake, great apes in zoological institutions are prone to obesity, potentially inducing several other adverse effects on the cardiovascular system, as discussed below.
Fibre and carbohydrates in the natural diet
As already mentioned, free-ranging great apes have very seasonal feeding patterns, whereby fruit-rich diets are never consumed throughout the whole year(Reference Hicks, Lee and Couto-Rodriguez75). Cabana stated that, to date, the term frugivore has led to two major misconceptions in zoological settings: great apes need high quantities of fruit the year round, and high proportions of soluble carbohydrates are required to maintain optimal health(Reference Cabana, Jasmi and Maguire76). Importantly, consumed fruits in their wild diets contain a completely different nutritional composition in comparison with fruit cultivated for human consumption, whereby the main difference is the high fibre and low soluble carbohydrate concentrations in wild-growing fruits(Reference Popovich, Jenkins and Kendall77). Following percentages of neutral detergent fibre (NDF) will be discussed on dry matter basis. For example, analysis of fruits consumed by wild chimpanzees showed estimated average NDF concentrations of 33·6–46·8%, which approaches NDF in other consumed plant parts such as leaves and pith(Reference Wrangham, Conklin and Chapman78,Reference Conklin-Brittain, Wrangham and Hunt79) . Similar studies, conducted in other free-ranging species such as western lowland gorillas, revealed that fibre contents of consumed fruits are even higher (64·6–78·7% NDF)(Reference Popovich, Jenkins and Kendall77,Reference Calvert80) . The seasonal influence in available wild-growing fruits was studied in orangutans, whereby in periods of favoured fruits abundance, the mean NDF concentration of consumed fruits is 28·7%, which differs greatly from periods wherein fruits are scarce and more fibrous (62·2% NDF)(Reference Knott81). The observed preference for less fibrous and more energy-dense fruits implies that orangutans, but also other great ape species in zoological settings, consume as much energy-dense fruit as possible, as an inherited energy storing strategy for use in seasons of ‘low-quality’ nutritional uptake(Reference Knott81,Reference Piel, Strampelli and Greathead82) .
Annual mean fibre consumption in great apes under human care lays in the range of 10–20% NDF, which is markedly low in comparison with the estimated minimal fibre contribution, of 50%, in the diet of free-ranging animals(Reference Cabana, Jasmi and Maguire76,Reference Remis and Dierenfeld83–Reference Less, Lukas and Bergl85) . Nutrient guidelines for captive apes proposed a NDF percentage of 10–30% with the remark that these values are not intended as maximal but represent achievable and rational guidelines for zoos(86). Reaching diet NDF concentrations of 50% is very complex in a zoo setting and is considered as the main reason why current guidelines do not correspond with the high fibre concentration consumed in the wild. Although the importance of fibre-rich diets gained more attention during recent years, many zoos still feed large amounts of commercial fruit and/or primate kibble. NDF percentages (20–26%) of such primate kibble fall far below these of wild diets. Therefore, diets presented to great apes in most zoos are often too low in fibre and abundant in sugars and starch(Reference Schmidt and Shaw12,Reference Smith, Remis and Dierenfeld87) . Such diets allow the animals to select the most energy-dense ingredients, which tends to cause long-term addiction to sugary foods, for example, reflected by fruit cravings(Reference Plowman88). Furthermore, significant quantities of commercial primate kibble also serve as an important source of energy in the form of amylopectin, a rapidly digestible starch(52).
Obesity is without question a detrimental consequence of an inappropriate diet, as energy-rich diets (i.e. high in fruit and low in browse) are likely to cause gradual increases in body weight in great apes, both in the wild and under human care(Reference Schmidt and Shaw12,Reference Smith, Remis and Dierenfeld87) . Transition to ‘fruit-free diets’ in great apes has led to interesting observations in group-fed conditions as most obese animals lost weight whilst other, originally lean animals, maintained their body weight. The most logical explanation for this phenomenon is that most dominant individuals can no longer gather energy-dense fruit, which is the most desired food item. Consequently, eliminating commercial fruit from great ape diets, primarily affects the weight of the most dominant individuals, who are often male and are particularly prone to developing CVD(Reference Plowman88).
Moreover, both soluble carbohydrates (fruit) and amylopectin starch (kibble) have high glycaemic indices, leading to high peaks of insulin secretion, promoting hyperinsulinaemia, which eventually may result in insulin resistance in the longer term(Reference Less, Lukas and Bergl85,Reference Plowman88,Reference Brand-Miller, Holt and Pawlak89) . Indeed, different authors concluded that exclusion of commercial fruit and/or primate kibble in combination with increasement of fibre content, leads to significant beneficial effects on the health of great apes(Reference Cabana, Jasmi and Maguire76,Reference Less, Lukas and Bergl85,Reference Cassella, Mills and Lukas90) . Cabana et al. (2018) noted that fasting blood glucose concentrations of orangutans and chimpanzees were reduced to normal, after diets were slowly decreased in carbohydrate and increased in fibre fractions(Reference Cabana, Jasmi and Maguire76). In a similar study, the effects of different diets on the insulin levels of gorillas were investigated. The lowest insulin levels were observed when gorillas were fed a kibble-free diet in combination with resistant starch sources, which require fermentation(Reference Less, Lukas and Bergl85).
To approach a high fibre content in zoological diets, 85–90% of great ape diets should exist of browse, leafy tree parts and low-starch vegetables(Reference Schmidt and Shaw12). Using browse as a fibre source also increases the duration of feeding and foraging without adding carbohydrates or starch as extra energy. For example, chimpanzees of the Taï National Park, Ivory Coast, spend approximately 54% of their daytime activities on foraging and eating(Reference Boesch and Boesch-Achermann91). Longer foraging time simulates this natural feeding behaviour and is associated with decreasing incidence of regurgitation and reingestion, an abnormal behaviour typical for primates under human care(Reference Cassella, Mills and Lukas90,Reference Struck, Videan, Fritz and Murphy92) . Moreover, an increased fibre (NDF) fraction in the diet of chimpanzees under human care significantly stimulated daily activity, foraging, and investigative and social affiliative behaviours(Reference Cabana, Jasmi and Maguire76). This also implies that energy expenditure increases, especially if the environment stays varied and challenging, which contributes to the maintenance of a normal body weight(Reference Cox, Carlsen, de Jongh and Pluháčková56).
Obesity and the effect on systolic blood pressure, endocrine signalling and lipid metabolism in great apes
Obesity in great apes housed in zoological institutions is a widely recognised problem(Reference Schmidt and Shaw12,Reference Ely, Zavaskis and Lammey93,Reference Videan, Fritz and Murphy94) . Despite numerous related comorbidities, little research has been done on establishing objective criteria to assess body condition in great apes, which resulted in the lack of knowledge about the incidence of obesity in zoos. Some authors reported that captive primates tend to weigh more than their free-ranging counterparts(Reference Smith, Remis and Dierenfeld87,Reference Leigh95,Reference Andrade, Higgins and Mattern96) . Objective criteria for obesity were defined only in chimpanzees, where waist circumference measurement was a reliable method in both sexes and BMI and skinfold measurements were decent tools in females but not in males(Reference Lowenstine, McManamon and Terio7,Reference Videan, Fritz and Murphy94,Reference Leigh95) .
Andrade et al. (2011) determined that higher adiposity, reflected by waist circumference, showed positive correlations with both systolic and diastolic blood pressure and fasting glucose values in female chimpanzees, while triacylglycerol values were positively corelated with waist circumference in both sexes(Reference Andrade, Higgins and Mattern96). In a similar study, comparable results were obtained, as abdominal skinfold measurements significantly correlated with both triacylglycerol and glucose values in female chimpanzees(Reference Videan, Fritz and Murphy94). In addition, a year of controlled reduction in caloric intake resulted in decreased abdominal skinfold thickness, systolic blood pressure and triacylglycerol and glucose values(Reference Videan, Fritz and Murphy94). The study by Ely and colleagues (2011) found other evidence suggesting that obesity increases systolic blood pressure in chimpanzees (n = 231). Although body weight is an inferior variable compared with waist circumference and skinfold measurements, chimpanzees in the bottom quartile (128 ± 2·2 mmHg) had a significantly lower systolic blood pressure than all three higher weight categories combined (143 ± 1·5 mmHg)(Reference Ely, Zavaskis and Lammey27). In a similar study from the same authors blood pressure measurements were performed in 201 chimpanzees, for 4 years, using a standardised protocol. Significant differences were observed between systolic but not diastolic blood pressure values of healthy and obese females. Obesity was defined by the combination of five different criteria, including abdominal circumference, body weight, BMI, body surface area and basal metabolic rate. The group of lean females had a median systolic blood pressure of 128 mmHg, in contrast to the median systolic blood pressure of 140 mmHg in overweight or obese females. For comparison, none of the measures of obesity significantly affected the systolic blood pressure of male individuals, but the values were noticeably higher with a median of 147 mmHg(Reference Ely, Zavaskis and Lammey93).
Although no data have been published examining the difference in criteria of obesity between great apes in zoos and in the wild. Comparisons have been made of plasma cholesterol and triacylglycerol levels between free-ranging and gorillas and orangutans under human care by Schmidt and colleagues (2006)(Reference Schmidt, Ellersieck and Cranfield97). In this study, samples were taken from free-ranging animals and plasma cholesterol and triacylglycerol values were compared with the mean concentrations derived from the international database for animals housed in zoos (Species360). The authors noted that total cholesterol and low-density lipoprotein cholesterol concentrations in gorillas in human care were significantly higher than in free-ranging animals. Moreover, triacylglycerol values from gorillas in human care were significantly higher than those observed in male free-ranging counterparts, but no significant differences were observed between female gorillas. For orangutans only a significant difference was observed between total cholesterol concentrations in female orangutans, whereas low-density lipoprotein cholesterol concentrations were significantly higher in both male and female orangutans in human care(Reference Schmidt, Ellersieck and Cranfield97). As previously mentioned, CVD in great apes is not associated with atherosclerosis by deposition of cholesterol plaques in the arterial blood vessel wall, and therefore the role of cholesterol as a direct actor on the development of cardiac fibrosis in great apes can be questioned(Reference Varki, Anderson and Herndon20). On the contrary, not high but lowered cholesterol values were significantly associated with echocardiographic parameters of cardiac disease in male gorillas (n = 44)(Reference Dennis, Raghanti and Meindl98).
However, the observed disturbances in cholesterol levels between wild and zoological situations and between lean and obese animals might support the hypothesis that great apes, like humans, are susceptible to the metabolic syndrome due to long-term improper nutrition. The metabolic syndrome is defined as the presence of at least three of the following physiological disturbances: obesity, insulin resistance (hyperinsulinaemia, hyperglycaemia), dyslipidaemia (elevated triacylglycerol and reduced high-density lipoprotein cholesterol) and systemic hypertension(Reference McCracken, Monaghan and Sreenivasan99). Each component of the metabolic syndrome can independently affect cardiac structure and function, but together these factors work in a synergistic way, as discussed below(Reference Voulgari, Tentolouris and Dilaveris100).
Obesity, the metabolic syndrome, oxidative stress and the repercussions on the cardiovascular system
Initiation of a disrupted endocrine signalling cascade in humans, as well as more recently observed in great apes, is attributed to obesity coupled with dysregulation of adipocytokine (adipokine) synthesis. Macrophages, which infiltrate adipose tissue, are involved in augmented NADPH oxidase and elevated reactive oxygen species (ROS), causing chronic low-grade inflammation(Reference Fernández-Sánchez, Madrigal-Santillán and Bautista101). Oxidative stress, reflected by the action of ROS, reduces the bioavailability of nitric oxide (NO). NO plays an important role in the regulation of the vascular tone. In the first instance, vascular resistant increases as a consequence of disruption of the NO-mediated vasodilatory tone(Reference Fernández-Sánchez, Madrigal-Santillán and Bautista101,Reference Vaziri and Rodríguez-Iturbe102) . Secondly, NO deficiency leads to arterial wall thickening due to vascular smooth muscle cell proliferation, matrix accumulation and vascular remodelling(Reference Vaziri and Rodríguez-Iturbe102). Last but not least, reduction of NO-mediated inhibition of central sympathetic outflow results in increased adrenergic vascular tone, which in turn is associated with RSNA and subsequent salt sensitivity (as mentioned above)(Reference Vaziri and Rodríguez-Iturbe102,Reference Ahmad, Dempsey and Daneva103) .
Increased oxidative stress and inflammation in visceral adipose tissues is the main cause of pro-inflammatory adipokine secretion in humans, including TNF-α, IL-6, IL-1β, MCP-1 and Ang II(Reference Furukawa, Fujita and Shimabukuro104). Interestingly, Nehete and colleagues (2014) confirmed these observations in chimpanzees, as obesity was associated with simultaneous induction of several pro-inflammatory cytokines (i.e. IFN-γ, IL-6, IL-12p40, TNF, sCD40L and IL-1γ) and metabolic hormones (i.e. insulin and leptin) in plasma of overweight (n = 10) and obese (n = 10) chimpanzees compared with lean (n = 28) counterparts(Reference Nehete, Magden and Nehete105). Secretion of pro-inflammatory factors, such as TNF-α and IL-6, leads to phosphorylation of insulin receptor substrate 1 (IRS-1), which disrupts normal insulin action in adipocytes and myocytes and are therefore important contributing factors in the development of insulin resistance(Reference DeMarco, Aroor and Sowers106). A lesser known but important function of insulin in humans is the regulation of the microvasculature tone as a key mechanism in the modulation of nutrient supply to the tissue. The action of insulin on the vascular tone consists of a delicate balance between vasodilatory endothelial NO production and secretion of the vasoconstrictor endothelin-1 (ET-1). During an insulin-resistant state, like obesity, the balance is shifted to upregulation of the ET-1 pathway and downregulation of the NO pathway, resulting in endothelial dysfunction and vascular resistance, facilitating systemic hypertension(Reference Muniyappa and Sowers107). Cardiomyocyte specific impaired insulin signalling also leads to similar structural cardiac abnormalities as observed in great ape hearts including collagen deposition and consequently interstitial and perivascular fibrosis, ventricular hypertrophy and sclerosis of the small coronary vessels(Reference Jia, DeMarco and Sowers108). Different authors stated that impaired myocardial insulin signalling leads to reduced activation of endothelial NOS and reduced levels of bioavailable NO parallel with elevations in oxidative stress(Reference Falcão-Pires and Leite-Moreira109,Reference D’Souza, Howarth and Yanni110) . Increases in ROS and linked inflammation, in combination with decreases in bioavailable NO, promote profibrotic TGF-β signalling, resulting in excessive collagen deposition, and crosslinking, which is associated with fibrosis and impaired myocardial function(Reference Jia, DeMarco and Sowers108). However, Dennis and colleagues were not able to confirm these hypotheses as high glucose, insulin and triacylglycerol levels were not associated with echocardiographic measures of heart thickness in gorillas(Reference Dennis, Raghanti and Meindl98). However, myocardial fibrosis precedes the development of structural cardiac changes, and therefore microscopic changes might have been missed(Reference Lowenstine, McManamon and Terio7,Reference Strong, Moittié and Sheppard17) . Therefore, a search for associations between different markers of metabolic syndrome and histopathological evidence of myocardial fibrosis is warranted to draw more nuanced conclusions.
Furthermore, as high leptin is indicative of increased adiposity in both chimpanzees and gorillas, a potential parallel with human obesity and CVD can be suggested(Reference Less, Lukas and Bergl85,Reference Nehete, Magden and Nehete105) . Indeed, high leptin and low adiponectin was also significantly and positively correlated with echocardiographic parameters of cardiac disease in male (n = 44) but not in female (n = 25) lowland gorillas under human care, in the study by Dennis and colleagues(Reference Dennis, Raghanti and Meindl98). This indirectly suggests that obesity may be linked to cardiovascular pathology in great apes. Noteworthy, hyperleptinaemia itself can raise systemic blood pressure by activation of the sympathetic nervous system, resulting in increased RSNA and sustained blood pressure elevations in humans(Reference da Silva, do Carmo and Li111). Remarkably, in contrast to obese states, chronic administration of leptin to lean rodents resulted in only a limited increase in systemic blood pressure(Reference Shek, Brands and Hall112). Kuo et al. (2001) noted that disruption of NO synthesis is the responsible factor in intensifying leptin-mediated hypertensive actions in obese individuals(Reference Kuo, Jones and Hall113). Ang II is another factor expressed by adipocytes in response to local adipose inflammation, resulting in higher circulating levels of Ang II in obese states(Reference Boustany, Bharadwaj and Daugherty114). As described above, Ang II has substantial hypertensive properties by mediation of vascular and renal vasoconstriction, NOS depletion and renal sodium reabsorption(Reference Mehta and Griendling115,Reference Husain, Hernandez and Ansari116) .
Comparison of vitamin D status in great apes: wild versus zoos
Over the past decade, fundamental research has taught us that vitamin D has protective properties against the development of myocardial fibrosis by modulating several of the pro-fibrotic pathways mentioned above. In addition, recent evidence showed that great apes housed in more northern regions are often vitamin D insufficient(Reference Videan, Heward and Fritz13–Reference Moittié, Jarvis and Bandelow15). These insights led to the hypothesis that vitamin D could be an important modulating factor in the development of CVD in great apes.
CVD has long been known to be associated with seasonality and latitude in humans, introducing a possible link between CVD and vitamin D deficiency(Reference Scragg117). The difference in the prevalence of myocardial fibrosis between some free-ranging great ape species and their counterparts under human care also corroborates this hypothesis. There are two ways in which primates can derive vitamin D, via oral uptake and via UV-B-radiation-mediated synthesis. Nutritional vitamin D can consist of two forms, namely plant-derived ergocalciferol (vitamin D2) and animal derived cholecalciferol (vitamin D3), both produced in living organisms by photosynthesis. Cholecalciferol has a much higher potency in raising and maintaining serum vitamin D concentrations in humans, making vitamin D3 the preferred form when correcting vitamin D deficiency(Reference Heaney, Recker and Grote118). Most food sources, except oily fish, liver and egg yolk, contain low doses of vitamin D3, and therefore a balanced diet will not provide adequate daily amounts of cholecalciferol and ergocalciferol to maintain a correct vitamin D status(Reference Pludowski, Holick and Grant119). This implies that the almost exclusively vegetarian diets of great apes are very low in the more bioactive vitamin D3 and mainly consist of vitamin D2-rich components. Therefore, a second pathway via UV-B-radiation-mediated synthesis in the skin is considered as the main source of vitamin D in great apes(Reference Christakos, Dhawan and Verstuyf120).
Most knowledge about the vitamin D status in great apes is derived from observational studies in chimpanzees; therefore, the following discussion will focus on this species. Genotyping studies show that the European captive chimpanzee population mainly (40%) consists of animals from West African origin, followed by 18% of Central African origin and 5% of East African origin. A big part of the captive population (21%) are hybrids with West African ancestry(Reference Hvilsom, Frandsen and Borsting121). Because of their equatorial distribution, UV indexes in these areas are often higher than 10 and rarely drop below 7(Reference Wright, Norval and Summers122). It is difficult to determine the main daily UV exposure, but this can be high. For example, chimpanzees of the Budongo Forest in Uganda easily stay about hundred minutes in the sunlight with temperatures approximately 28–30 °C(Reference Kosheleff and Anderson123). Also, the habitat can influence daily UV exposure. There are different kinds of habitats where wild chimpanzee populations can be found, ranging from dense tropical rain forests in Tai National Park, Ivory Coast(Reference Boesch and Boesch-Achermann124), open deciduous forests and woodland in Gombe National Park, Tanzania(Reference Collins and McGrew125), swamp forests in Northern Congo(Reference Poulsen and Clark126) to grassland with only scattered trees in Mt. Assirik, Senegal(Reference McGrew, Baldwin and Tutin127). It is interesting to note that even within the subspecies of the West African chimpanzee, as an example for zoo populations, the habitat varies from rainforest to open, dry savannah area. This means that the average of UV exposure between populations in other habitats can differ. This suggests that also vitamin D levels between these populations will show fluctuations.
To establish reference values, measurements in wild chimpanzees living in their natural habitats and consuming their natural diets, must be done(Reference Junge, Gannon and Porton128). The assumed fluctuations will make it difficult to establish reference values. The second problem is the absence of available serum 25-hydroxy vitamin D (25(OH)D) values from wild chimpanzees (of note, 25(OH)D is the circulatory form of vitamin D and commonly used to determine the vitamin D status of animals). The only 25(OH)D values that can be compared with those of wild chimpanzees, were obtained from animals in local rescue centres located in Angola, Guinea-Bissau and Rwanda. The mean 25(OH)D value of these animals (n = 14) was 118 ± 47 nmol/l(Reference Janssens, van Noije and Kaandorp14). This information gives an indication of normal 25(OH)D levels in chimpanzees. Yet, because of the small number of samples and the fact that these animals are not living in a natural habitat, valid reference values cannot be defined. The most used reference value for chimpanzees and other primates originates from human standards. The European guidelines nowadays recommend maintenance of serum 25(OH)D concentrations in the range of 75–125 nmol/l(Reference Pludowski, Holick and Grant119). It is noteworthy that the mean value of animals (n = 14) in local shelters was within this range(Reference Janssens, van Noije and Kaandorp14).
Chimpanzees kept in more northern regions are exposed to lesser UV radiation. For example, in most West-European countries the UV index from October to March lies between 3 and 6. In addition, some zoo infrastructures do not provide outside access. To overcome vitamin D deficiency in chimpanzees, housed in zoos, several recommendations have been generally accepted. These mainly include unrestricted outdoor access and oral vitamin D supplementation(Reference Videan, Heward and Fritz13,Reference Elliott, Walker and Little54,Reference Junge, Gannon and Porton128–Reference Steinmetz, Redrobe, Potier, Carlsen, de Jongh and Pluháčková130) .
The proposed nutrient guideline for primates, in absence of solar or artificial UV-B radiation, states a dietary vitamin D3 concentration of 1000–3000 IU/kg on dry matter basis(129). However, obtaining these values is not feasible without supplementation in the recommended high-fibre/low-kibble diets. Especially in young chimpanzees, oral vitamin D3 supplementation with human preparations, for breastfed infants, is recommended to support appropriate bone growth and development(Reference Schmidt and Shaw12). This is due to known problems of rickets in young animals who were housed exclusively indoors(Reference Junge, Gannon and Porton128). Due to the following data, questions may be raised about the above recommendations: the mean 25(OH)D value of different primate species was measured during a retrospective study over two years in different zoos located in the United States(Reference Crissey, Barr and Slifka131). The sampled animals had opportunities of daily exposure to UV radiation through natural sunlight. The nutrient requirements of these animals were based on the non-human primate guidelines, and the diets met the accepted vitamin D3 levels with a minimum of 2800 IU/kg(Reference Cox, Carlsen, de Jongh and Pluháčková56). Still, following the recommendations, chimpanzees (n = 14) had the lowest 25(OH)D serum levels of all measured species, with a mean value of only 32·7 ± 3·5 nmol/l(Reference Crissey, Barr and Slifka131). Another study by Videan and colleagues showed that, despite of a balanced diet and daily average dietary intake of 3000 IU vitamin D3 (with supplementation), female chimpanzees (n = 18) showed a significant decrease from 53·7 to 38·2 nmol/l in serum 25(OH)D values after a six-month period of abstinence from sunlight(Reference Videan, Heward and Fritz13). The recommended dietary intake of vitamin D can therefore not be considered an alternative to daily sunlight exposure and even with access to natural sunlight, vitamin D levels in the studied populations are generally low.
Indeed, it has recently been confirmed that seasonal vitamin D insufficiency is widespread in chimpanzees housed in European zoos, as 33·1% of the tested population (n = 245) had serum 25(OH)D levels lower than 50 nmol/l(Reference Moittié, Jarvis and Bandelow15). Also in this population, additional oral vitamin D3 supplementation did not significantly influence serum 25(OH)D concentrations. Interestingly, in the subgroup with unrestricted outdoor access, on the other hand, 25(OH)D levels were on average 19·9 nmol/l higher. Noteworthy, only twenty-one animals received oral vitamin D3 supplements, demonstrating the relative ignorance of zoos about possible vitamin D insufficiency in great apes. Fluctuations in serum vitamin D concentrations also occur due to seasonal changes. For example, compared to the summer season, 25(OH)D concentrations were 25·5 nmol/l lower in winter(Reference Moittié, Jarvis and Bandelow15). Our group reported similar observations, whereby the highest mean 25(OH)D values were seen in summer (n = 19, 83 ± 36 nmol/l) with a decline during autumn (n = 22, 71 ± 33 nmol/l) and the lowest values were measured in winter (n = 13, 49 ± 33 nmol/l). In this retrospective study, the mean 25(OH)D value of chimpanzees, with correct supplementation, was 64·8 ± 29 nmol/l(Reference Janssens, van Noije and Kaandorp14). In the study by Moittié et al. the percentage of samples that fell below the threshold of 50 nmol/l per season was 55·1% in winter, 28% in spring, 23·4% in summer and 31·3% in autumn. Even chimpanzees housed in Southern European zoos were not always able to maintain an adequate vitamin D status, as 16% of deficient samples came from such animals(Reference Moittié, Jarvis and Bandelow15).
No direct clinical signs of vitamin D deficiency have been observed in adult animals under human care. Also, serum calcium and phosphorus levels from chimpanzees (n = 8), with no exposure to sunlight or artificial UV radiation, were considered normal. These animals had a mean serum 25(OH)D value of 31 nmol/l, but in this group three infants were diagnosed with symptoms of rickets. On radiography, skeletal abnormalities were seen in a 4-month-old chimpanzee with a serum 25(OH)D value of 31·9 nmol/l(Reference Junge, Gannon and Porton128). In human medicine, the minimal value for rickets prevention is 25 nmol/l(Reference Spedding, Vanlint and Morris132). Interesting to note is that this human reference value did not apply for this 4-month-old chimpanzee. This may also indicate that serum levels of approximately 30 nmol/l can give visible bone pathology in infants, but not in older animals.
Different conclusions can be drawn from the information above. All analysed serum 25(OH)D concentrations from chimpanzees housed in zoos lay far below the mean concentration (118 nmol/l) of animals in local rescue centres, and most individual animals did not reach the minimal human 25(OH)D reference value of 75 nmol/l for pleiotropic effects. Especially in animals with no access to UV radiation, due to indoor housing or during winter, 25(OH)D levels can drop easily. The gradual decrease is present even despite following dietary advice. This raises the question whether higher supplementation doses can support the maintenance of adequate vitamin D status in this species. A final observation is that in some studies serum 25(OH)D levels are low even at the recommended UV exposure due to outdoor access. This indicates that higher UV indices and/or longer daily exposure are likely required to maintain an adequate vitamin D status(Reference Steinmetz, Redrobe, Potier, Carlsen, de Jongh and Pluháčková130).
Vitamin D and cardiovascular disease
Multiple cells of the cardiovascular system carry the vitamin D receptor (VDR), including cardiomyocytes, endothelial cells and vascular smooth muscle cells, and it is well established that vitamin D receptor knockout is associated with the development of myocardial hypertrophy and dysfunction(Reference Gonzalez-Parra, Rojas-Rivera and Tuñón133–Reference Pilz, Verheyen and Grübler135). Furthermore, numerous fundamental studies have already proven several beneficial effects of vitamin D on the cardiovascular structure and function(Reference Nizami, Katare and Prabhakar136–Reference Mancuso, Rahman and Hershey144). Some of the underlying mechanisms have already been elucidated, as summarised in Table 1.
Table 1. Summary of rodent models demonstrating underlying mechanisms of the preventive role of vitamin D in the development of myocardial fibrosis and dysfunction

Numerous human epidemiological studies confirmed an association between serum 25(OH)D values and cardiovascular risk factors and diseases. For example, a large observational study evaluated the incidence of acute myocardial infarction in 18 000 men, which was 2·42 times higher in men with 25(OH)D levels below 37·5 nmol/l compared with those with levels above 75 nmol/l(Reference Giovannucci, Liu and Hollis145). In a systematic review and meta-analysis of observational studies (101 649 individuals) comparing bottom versus top thirds of baseline circulating 25(OH)D values, pooled relative risks for cardiovascular-related deaths were 1·35(Reference Chowdhury, Kunutsor and Vitezova146). Another meta-analysis of eight cohort studies, consisting of 23 081 subjects, pointed out the association between the bottom 25(OH)D quintile and increased cardiovascular mortality, reflected by a relative risk of 1·41(Reference Schöttker, Jorde and Peasey147).
However, vitamin D deficiency is associated not only with cardiovascular but also with all-cause mortality, indicating that vitamin D deficiency might solely be a characterisation of poor general health(Reference Pilz, Tomaschitz and Drechsler134,Reference Schöttker, Jorde and Peasey147) . Interestingly, also in chimpanzees, poor health status was associated with a decrease in vitamin D levels(Reference Moittié, Jarvis and Bandelow15). Therefore, some research groups assume that many disease states will reduce activity and thus sun exposure, leading to reverse causality(Reference Christakos, Dhawan and Verstuyf120). This hypothesis was approved in a large, randomised placebo-controlled trial with a total of 25 871 participants, in which 5 years of supplementation with vitamin D3, at a dose of 2000 IU/d, did not lead to a significantly lower incidence of cardiovascular events(Reference Manson, Cook and Lee148). An important remark to this study is that the mean age of the subjects was 67 years with a percentage of 50% and 38% of participants treated with antihypertensive or cholesterol-lowering medication, respectively. This implies that cardiovascular degenerative processes might have reached advanced stages before the onset of the study, pointing out that, despite vitamin D3 administration, the ongoing processes were irreversible. Interestingly, vitamin D deficiency during childhood is a major risk factor for the development of CVD during adulthood(Reference Pludowski, Holick and Grant119,Reference Juonala, Voipio and Pahkala149) . Children aged 3–18 years (cohort of 2148 participants) with 25(OH)D levels in the lowest quartile (<40 nmol/l) had significantly increased odds of having high-risk carotid intima-media thickness as adults, a marker correlated with future cardiovascular events. Adjustments were made for age, sex and childhood risk factors (odds ratio 1·70) or adult risk factors, including adult serum 25(OH)D levels (odds ratio 1·80)(Reference Juonala, Voipio and Pahkala149). Therefore, it can be deduced that an adequate vitamin D status may have a preventive effect against the onset of cardiovascular disease but has little or no effect on reversing marked cardiovascular degeneration.
Strong et al. (2020) investigated possible causative factors for interstitial myocardial fibrosis in a zoo-housed chimpanzee population. Interestingly, post-mortem examinations revealed that the worst cases of interstitial myocardial fibrosis, leading to sudden cardiac death, were accompanied by significant vitamin D deficiencies. For example, the animal affected with the most severe myocardial fibrosis even had inadequate levels of serum 25(OH)D during summer (45·4 nmol/l) and died after the subsequent winter(Reference Strong, Moittié and Sheppard17).
Retrospective data analysis showed that sampled primates, with a higher degree of skin pigmentation, had significantly lower serum 25(OH)D values(Reference Janssens, van Noije and Kaandorp14). This implicates that especially primates with more skin pigmentation are more sensitive to vitamin D deficiency, caused by the protective effect of skin pigment against UV radiation(Reference Hagenau, Vest and Gissel150). To date, however, no data have been published on possible associations between skin pigment scores and the risk of developing CVD in great apes. Remarkably, the only chimpanzee with no histopathological signs of myocardial fibrosis, in the study by Strong and colleagues, had significantly higher serum levels of 25(OH)D (182·9 nmol/l). The most logical explanation for these comparatively higher serum values lies in the paler skin pigmentation and the full body alopecia, which characterised the animal(Reference Strong, Moittié and Sheppard17). More epidemiological data and well-designed interventional studies are required to determine whether vitamin D deficiency is a major contributing factor to cardiac fibrosis in great apes.
Summary and conclusions
Diet compositions of great apes kept in zoological institutions differ considerably from these consumed in the wild. Excessive sodium intake, a carbohydrate–fibre mismatch and low vitamin D status are some of the most pivotal dietary imbalances in zoological conditions. Long-term inadequate nutrition in great apes has repercussions on various physiological processes.
Long-term excessive sodium uptake, primarily due to large amounts of commercial primate kibble, may result in a chronic hypertensive state. Both macroscopic (i.e. concentric left ventricular hypertrophy and intrinsic coronary artery hypertrophy) and microscopic (i.e. myocardial fibrosis) features of cardiovascular disease in great apes indicate that hypertension is in all probability an important underlying factor. Hypertension is likely to be exacerbated in animals with reduced sodium excretion capacity. As in humans, chronic renal insufficiency, a major cause of decreased sodium excretion and hypertension, is associated with cardiovascular disease in great apes. Furthermore, a significantly shorter foraging time when feeding high-energy diets disrupts natural feeding behaviour, leading to boredom and subsequent stress. Chronic stress, reflected by a higher sympathetic state, may also worsen systemic hypertension, at least in part due to an increase in renal vascular tone with a consequent negative effect on sodium excretion.
In the wild, these species mainly consume foods that are low in simple carbohydrates and high in fibre, which is also reflected by their high capacity of hind-gut fermentation. Feeding energy-dense fruits and primate kibble is one of the main reasons why these animals develop obesity in zoos. Significantly higher systolic blood pressures are often detected in more obese animals. In addition to hypertension and obesity, impaired insulin signalling and dysregulation of lipid metabolism are also commonly observed in zoological populations of great apes. These observations indicate that an equivalent of the human metabolic syndrome may be present in these species. As in humans, impaired insulin signalling is likely present due to increased low-grade inflammation and oxidative stress secondary to increased adiposity. To date, however, there is no direct evidence showing that insulin resistance contributes to myocardial fibrosis in great apes. Moreover, increased cholesterol values are not associated with cardiovascular disease in great apes. This is consistent with the observation that coronary artery atherosclerosis is completely absent in great ape hearts.
Despite following dietary intake advice, serum vitamin D (25(OH)D) levels of animals kept in more northern regions are low, even with recommended UV exposure due to outdoor access. This suggests that higher UV indices and/or longer daily UV exposure are needed to maintain a correct vitamin D status. Recently, several pleiotropic effects of vitamin D on genes involved in oxidative stress and nitric oxide bioavailability have been discovered, implying an attenuation of profibrotic signalling. Moreover, animal models of pressure overload and salt sensitivity showed that vitamin D has a preventive role in the formation of myocardial fibrosis. A possible link between myocardial fibrosis and vitamin D deficiency was also hypothesised on the basis of recent observations in chimpanzees. Individual cases showed that more advanced fibrotic myocardial lesions were associated with low serum 25(OH)D levels and vice versa. As in humans, the cardioprotective role of vitamin D is still unclear and further research is necessary to demonstrate the influence of an adequate vitamin D status in the prevention of cardiovascular disease in great apes.
It is fascinating to observe that humans’ closest relatives share a high prevalence for hypertension and cardiovascular disease. Although there is no recognised equivalent to the pattern of myocardial fibrosis observed in human patients, most dietary factors involved in the development of cardiovascular disease in humans also appear to underlie the disease process in great apes. The influences of chronic excessive sodium intake and obesity on systolic blood pressure can be considered the most important elucidated mechanisms. Seasonal low vitamin D status appears to be common in populations under human care, but its importance in cardiovascular disease development is still unclear and should form the subject of future research lines. Comprehension of the highlighted dietary aberrations warrants a more preventive approach to great ape cardiovascular disease management and paves the way for future clinical trials to gain a better understanding of the impact of each individual factor on the development of cardiovascular disease (Figs. 1 and 2).
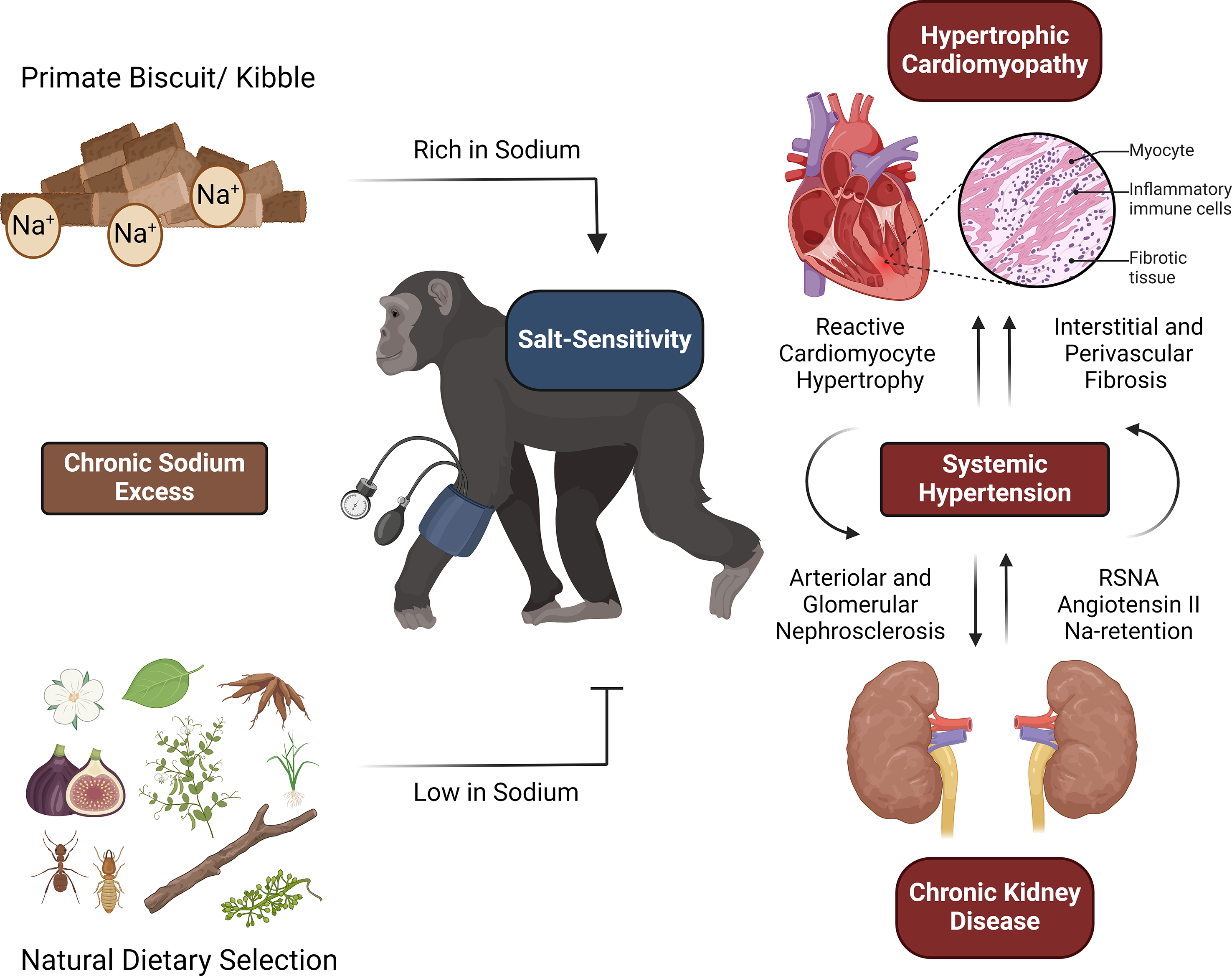
Fig. 1. Great apes may not be adapted to extended consumption of sodium-rich elements, given that their natural diets primarily consist of items with low sodium content. Therefore, long-term excessive sodium uptake, primarily due to large amounts of commercial primate kibble, can result in a chronic hypertensive state. Both macroscopic (i.e. hypertrophic cardiomyopathy) and microscopic (i.e. perivascular fibrosis) features of cardiovascular disease in great apes indicate that hypertension is in all probability an important underlying factor. Hypertension is likely to be exacerbated in animals with reduced sodium excretion capacity, a condition also referred to as salt sensitivity. As in humans, chronic kidney disease, a major cause of decreased sodium excretion and hypertension, is associated with cardiovascular disease in great apes RSNA, renal sympathetic nerve activity.

Fig. 2. In the wild, great ape species mainly consume foods that are low in complex carbohydrates and high in fibre. Feeding cultivated fruit and primate kibble often leads to a mismatch between caloric intake/expenditure, and is therefore one of the main reasons why these animals develop obesity in zoos. As in humans, impaired insulin signalling and nitric oxide depletion is likely present due to increased low-grade inflammation and oxidative stress secondary to increased adiposity. In combination with chronic stress, resulting from boredom associated with reduced feeding/foraging time, these processes promote systemic hypertension. Together, these phenomena may induce the formation of myocardial fibrosis in a synergistic way.
Acknowledgements
The authors would like to thank all researchers and members of the Great Ape Heart Project for their efforts in facilitating a rapid progress in cardiovascular disease research in great apes. From May 2023 onwards, Christine Kaandorp’s affiliations have changed, now including Diergaarde Blijdorp, Rotterdam, The Netherlands, and GaiaZoo, Kerkrade, The Netherlands.
Financial support
This research received no specific grant from any funding agency, commercial or not-for-profit sectors.
Competing interests
The authors declare that they have no conflict of interest.
Authorship
The main author of this review, Laurens Van Mulders, was primarily responsible for a thorough literature search in the context of this topic. In addition, he was assisted by all co-authors in delving into more specific subjects related to each co-author’s specific expertise. For example, Geert Janssens assisted with analysing specific research into nutrition-related themes, such as natural feeding habits of great apes, and helped indicate the discrepancies in nutritional components between natural and zoo diets and their potential impact on homeostatic physiological balances. Laurent Locquet contributed from his expertise in cardiology and his role in the European Great Ape Heart Project to literature analysis of clinical and pathophysiological data on cardiovascular disease and myocardial fibrosis. Due to her many years of experience in zoo medicine, Christine Kaandorp, helped with searching and reporting currently applied zootechnical data, such as current feeding and supplementation strategies/schedules in great apes under human care. All authors have read and revised multiple drafts of the review thoroughly before agreeing on the final version.