Discovery and Mode of Action of Protoporphyrinogen Oxidase (PPO)-inhibiting Herbicides
The first PPO-inhibiting herbicide patent was filed by Rohm and Haas in 1960 (issued in 1963) for the diphenyl ether (DPE) nitrofen (Wilson and McRae Reference Wilson and McRae1963). There are now 22 herbicides targeting PPO: 10 phenyl-imides (e.g., butafenacil), 5 DPEs (e.g., acifluorfen), 3 N-phenyl-triazolinones (e.g., sulfentrazone), 2 N-phenyl-oxadiazolones (e.g., oxadiazon), and 2 that belong to other chemical classes (Herbicide Resistance Action Committee [HRAC] 2022). PPO is a very promiscuous target, with more than 100,000 experimental molecules with inhibitory activity against this enzyme.
Initial research into the mode of action (MoA) of Group 14 herbicides had been confounded by the fact that various DPE compounds had different enzyme targets (Kunert et al. Reference Kunert, Sandmann and Böger1987), including PPO (Dayan and Duke Reference Dayan, Duke and Krieger2010), acetyl-CoA carboxylase (Zhang et al. Reference Zhang, Tweel and Tong2004), enoyl ACP reductase (McMurry et al. Reference McMurry, McDermott and Levy1998), and solanesyl diphosphate synthase (Kahlau et al. Reference Kahlau, Schröder, Freigang, Laber, Lange, Passon, Kleeßen, Lohse, Schulz and von Koskull-Döring2020). However, only PPO inhibitors cause rapid chlorosis, wilting, and necrosis. Because these symptoms are reminiscent of paraquat injury, some thought that these herbicides might become free radicals by in planta photoactivation (Orr and Hess Reference Orr and Hess1982). There was evidence for (Lambert et al. Reference Lambert, Kroneck and Böger1984) and against (Duke and Kenyon Reference Duke and Kenyon1986) the involvement of photosynthesis in DPE activity. A chlorophyll precursor (chlorophyllide) was proposed as a photodynamic pigment responsible for at least part of the activity of DPEs in 1987 (Duke and Kenyon Reference Duke and Kenyon1987). Based on symptoms, these herbicides were initially called “bleaching herbicides” or “peroxidizing herbicides” by many. Research with PPO-inhibiting herbicides ultimately concluded that light was required for activity, but the basis for this light requirement was unknown.
Later, Matringe and Scalla (Reference Matringe and Scalla1987) reported that acifluorfen-methyl causes accumulation of the chlorophyll and heme precursor protoporphyrin IX (proto) in plant tissues (Figure 1). They also demonstrated that the inhibitor of porphyrin synthesis, 4,6-dioxoheptanoic acid, prevented both accumulation of proto and herbicidal effects of acifluorfen-methyl. Theoretically, proto accumulation should be caused by inhibition of one of the downstream enzymes that channel proto to chlorophyll and heme, respectively (i.e., Mg-chelatase and/or Fe-chelatase) (Figure 1). However, these authors published data indicating that PPO was the target of these herbicides a year later (Matringe and Scalla Reference Matringe and Scalla1988). Similar findings from three other labs supported that breakthrough discovery (Lydon and Duke Reference Lydon and Duke1988; Sandmann and Böger Reference Sandmann and Böger1988; Witkowski and Halling Reference Witkowski and Halling1988). In fact, DPE herbicides inhibit PPO 2,000 to 100,000 times more than the two chelatases for which proto is the substrate (Matringe et al. Reference Matringe, Camadro, Labbe and Scalla1989). Clues pointing toward PPO came from unrelated studies showing that defects in PPO in humans and yeast lead to proto accumulation. Note that two of the authors of PPO target-site discovery paper were authors of one of these early papers on defective PPO in mammalian systems. They recognized that, as with the case of the human disease variegate porphyria caused by a defect in PPO, inhibition of PPO could cause the substrate, protoporphyrinogen (protogen), to diffuse out of the porphyrin pathway site to other parts of the cell.
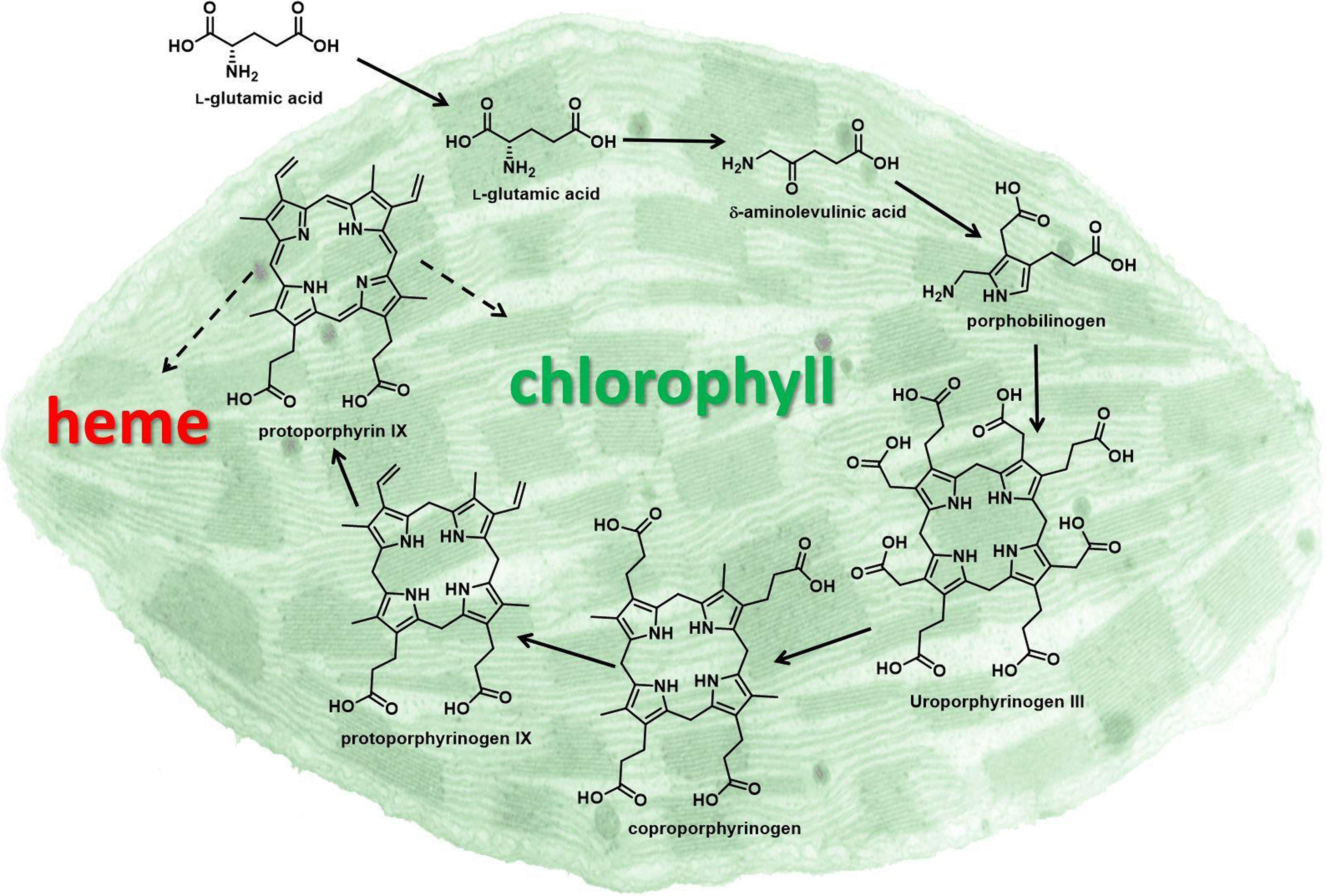
Figure 1. Porphyrin pathway of the chloroplast. Solid arrows indicate single enzymatic steps, and dashed arrows indicate multiple enzymatic steps. Protoporphyrinogen oxidase (PPO) catalyzes the enzymatic reaction converting protoporphyrinogen IX to protoporphyrin IX, the penultimate step in porphyrin biosynthesis.
Mechanism of Action
Proto is a photodynamic metabolic intermediate (Figure 1); therefore, its biosynthesis is tightly regulated to prevent proto accumulation, because it can generate singlet oxygen in the presence of light and molecular oxygen. When PPO is inhibited, protogen leaks out of the chloroplast and is converted to proto in the cytosol (Lehnen et al. Reference Lehnen, Sherman, Becerril and Duke1990; Figure 2). This is the physiological basis for the very rapid, light-dependent activity of PPO-inhibiting herbicides. Singlet oxygen generation leads to a cascade of reactive oxygen species (ROS) production, including lipid peroxides that cause loss of membrane function and integrity (Dayan et al. Reference Dayan, Barker, Dayan and Ravet2019). Rapid cellular leakage due to the loss of plasma membrane integrity is a hallmark symptom of DPE activity (Kenyon et al. Reference Kenyon, Duke and Vaughn1985). The accumulation of proto could only be found in dark-incubated plant tissues, because the proto-generated ROS in the presence of light also lead to destruction of proto. The initial half-life of dark-accumulated proto in vivo in DPE-treated cucumber (Cucumis sativus L.) cotyledons is only 2.5 h under the equivalent of one-quarter full sunlight (Becerril and Duke Reference Becerril and Duke1989a). Proto accumulation in plant tissues treated with different herbicides in darkness is strongly correlated with subsequent herbicidal damage when exposed to light, regardless of the plant species (Becerril and Duke Reference Becerril and Duke1989b). A summary of the steps involved in the mechanism of PPO-inhibiting herbicide action is described in Figure 3.
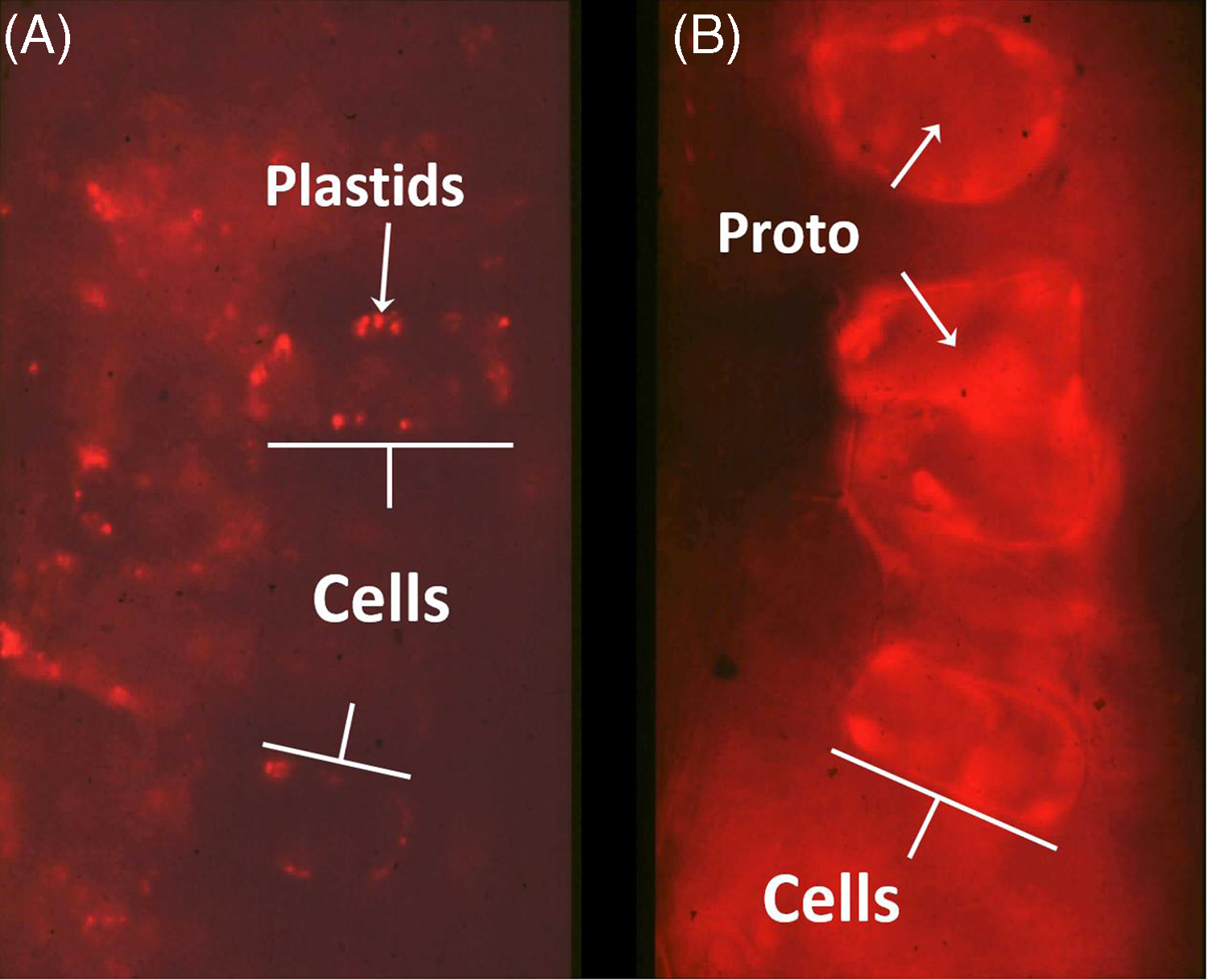
Figure 2. Fluorescence micrographs of tentoxin-treated (keeps chlorophyll from accumulating) cucumber cotyledon cells as a control (A) and with acifluorfen-methyl treatment (B). Note protoporphyrin IX (proto) accumulation along cell walls of acifluorfen-methyl-treated cells, indicating association with the plasma membrane. Unpublished images from the work of Lehnen et al. (Reference Lehnen, Sherman, Becerril and Duke1990).

Figure 3. Mechanism of action of protoporphyrinogen oxidase (PPO) inhibitors. In the case of postemergence applied herbicides, the active ingredient must penetrate the cuticle, cross the epidermis, and enter photosynthetically active parenchyma cells. The herbicide then inhibits PPO located in the outer envelope of the chloroplasts. This causes the colorless protoporphyrinogen (protogen) precursor to leak out into the cytoplasm, where it is converted to the highly photodynamic protoporphyrin IX (proto). In the presence of light, proto generates a burst of reactive oxygen species (ROS) that react with membrane lipid and cause lipid peroxidation.
Low doses of PPO inhibitors can also generate low levels of ROS that induce production of plant defenses against pathogens, which was previously reviewed (Duke Reference Duke2018). For example, the herbicide label for lactofen even recommends low rates (100 to 130 g ha−1) for suppression of white mold (Sclerotinia sclerotiorum deBary) in soybean [Glycine max (L.) Merr.]. It is sometimes used as a growth regulator to prevent soybean bending due to overgrowth.
The best proof of this conclusion is that plants expressing transgenes of herbicide-resistant PPO have high levels of resistance (up to 1,000×) to PPO-inhibiting herbicides (PPO-R) (Li and Nicholl Reference Li and Nicholl2005; Li et al. Reference Li, Volrath, Nicholl, Chilcott, Johnson, Ward and Law2003; Figure 4). Although crops with PPO-R traits reached the trade name stage, they have not been commercialized, perhaps because of the dominance of glyphosate-resistant crops at the time when they were being developed. Herbicide-tolerance traits for dicamba, 2,4-dichlorophenoxyacetic acid, isoxaflutole, mesotrione, glufosinate, and stacks thereof were developed and brought to market instead, as these herbicides were preferred by the seed and agrochemical industries involved. Recent publications and related patent applications indicate the development of herbicide-resistance traits based on waterhemp [Amaranthus tuberculatus (Moq.) Sauer] PPX2 mutations (Aponte et al. Reference Aponte, Mankin, Tresch, Lerchl, Witschel, Mietzner, Armel and Liebl2017) as well as the use of engineered bacterial hemG genes encoding a microbial PPO enzyme that is naturally tolerant to PPO-inhibiting herbicides (Larue et al. Reference Larue, Ream, Zhou, Moshiri, Howe, Goley, Sparks, Voss, Hall, Ellis, Weihe, Qi, Ribeiro, Wei and Guo2020) and that commercial level of resistance toward PPO inhibitors is feasible with these transgenes.

Figure 4. Effects of 400 g ha−1 of the protoporphyrinogen oxidase (PPO) inhibitor butafenacil (3× recommended field rate) on wild-type corn (left) and corn transformed with a herbicide-resistant PPO at 8 d after treatment (right). From Li et al. (Reference Li, Volrath, Nicholl, Chilcott, Johnson, Ward and Law2003) with permission.
History and Distribution of PPO-resistant Weeds
PPO-inhibiting herbicides were commercialized more than 50 yr ago (Dayan et al. Reference Dayan, Barker and Tranel2018) and used preemergence and postemergence in combinations with other herbicides. The introduction of glyphosate-resistant soybean and corn (Zea mays L.) in the late 1990s reduced farmers’ reliance on PPO inhibitors, as well as other herbicides. After several years of intensive use of glyphosate, the evolution and dramatic spread of glyphosate-resistant weeds, especially Amaranthus species, renewed interest in PPO inhibitors.
The first case of PPO-R involved A. tuberculatus resistance to fomesafen, lactofen, and acifluorfen (Heap Reference Heap2023; Shoup et al. Reference Shoup, Al-Khatib and Peterson2003). This population also had strong resistance to several acetolactate synthase (ALS) inhibitors (HRAC Group 2). As of 2022, 40 unique cases of PPO-R weeds were described, 35 in dicotyledonous weeds and 5 in monocotyledonous weeds (Table 1). Interestingly, 23 of these cases involve Amaranthus spp.
Table 1. Number of unique resistance cases to protoporphyrinogen oxidase (PPO) inhibitors per weed species and country. a

a Data from International Herbicide-Resistant Weed Database (Heap Reference Heap2023).
These cases were discovered in eight countries (Table 1), 65% being in the USA, 73% in North America, and 15% in South America. As of 2022, 34 cases out of 40 (85%) were identified in soybean, corn, and/or cotton (Gossypium hirsutum L.), the remaining being in cereals and turf, suggesting that PPO-R can evolve in weeds growing in a broad range of crops.
It is striking that PPO-R is, with a high frequency, associated with resistance to herbicides with other MoAs (i.e., multiple-resistant cases) (Table 2). This might be due to: (1) the use of different herbicides in sequence or mixtures and independent evolution of resistance to compounds of each MoA; (2) the evolution of unspecific non–target site resistance (NTSR), such as metabolic resistance, conferring resistance to a broad range of different herbicide chemistries inhibiting different targets (Adhikari et al. Reference Adhikari, Goodrich, Fernandes, Lipka, Tranel, Brown and Jamann2020; Comont et al. Reference Comont, Lowe, Hull, Crook, Hicks, Onkokesung, Beffa, Childs, Edwards and Freckleton2020; Han et al. Reference Han, Yu, Beffa, González, Maiwald, Wang and Powles2021); or (3) a combination of both mechanisms. It is worth noticing that 29 cases of the 40 described are multiresistant, with 7 cases resistant to five or more MoAs (Table 3). PPO-R is often associated with resistance to glyphosate (inhibitor of 5-enolpyruvylshikimate-3-phosphate synthase [EPSPS]) (HRAC Group 9) and/or ALS inhibitors (HRAC Group 2) (Table 4). In addition, many of the 40 cases (Heap Reference Heap2023) also had resistance to herbicides with other MoAs, such as photosystem I (HRAC Group 22), photosystem II (PSII) (HRAC Groups 5 and 6), auxins (HRAC Group 4), carotenoid biosynthesis inhibitors (HRAC Group 12), acetyl-CoA carboxylase (ACCase) (HRAC Group 1), very-long-chain fatty-acid synthesis inhibitors (VLCFAs) (HRAC Group 15), microtubule inhibitors (HRAC Group 23), and 4-hydroxyphenylpyruvate dioxygenase (HPPD) inhibitors (HRAC Group 27).
Table 2. Number of unique cases of resistance to protoporphyrinogen oxidase (PPO) inhibitors, and whether the case involves a single resistance or multiple resistance profile. a

a Data from the International Herbicide-Resistant Weed Database (Heap Reference Heap2023).
Table 3. Number of unique protoporphyrinogen oxidase (PPO) inhibitor–resistant cases of resistance to one or several modes of action (MoAs). a

a Data from the International Herbicide-Resistant Weed Database (Heap Reference Heap2023).
Table 4. Number of unique cases resistant to protoporphyrinogen oxidase (PPO) inhibitors and glyphosate (EPSPS) and/or acetolactate synthase (ALS) inhibitors in different combinations. a

a Data from the International Herbicide-Resistant Weed Database (Heap Reference Heap2023).
Evolution of PPO-R over time is of interest (Figure 5). The rapid increase in the number of cases of PPO-R that occurred from 2013 to 2017 is largely attributable to a resurgence in use of PPO inhibitors to address widespread resistance to glyphosate and ALS inhibitors. The subsequent slowdown in new PPO-R cases since 2017 perhaps is due at least in part to widespread adoption of crops with resistance to dicamba or 2,4-D.

Figure 5. Evolution of the number of the unique cases of resistance to protoporphyrinogen oxidase (PPO) inhibitors from 2001 to 2021. Data from the International Herbicide-Resistant Weed Database (Heap Reference Heap2023).
Mechanisms of Resistance to PPO Inhibitors
As is the case with resistance to most other herbicides, both target site–resistance (TSR) and NTSR mechanisms exist for PPO inhibitors, but the NTSR mechanisms remain poorly understood. Of the 14 PPO inhibitor–resistant weed species listed by the International Herbicide-Resistant Weed Database (Heap Reference Heap2023), 6 have mutations imparting TSR. Before discussing these PPO mutations, however, it is important to first understand that there are two potential target site for PPO inhibitors.
Two PPO Enzymes for TSR
PPO activity is needed both for chlorophyll production in chloroplasts and for heme production in mitochondria. Consequently, there are two separate PPO enzymes that are encoded by the nuclear genes PPX1 and PPX2 (Dayan et al. Reference Dayan, Barker and Tranel2018). Traditionally, it was thought that PPX1 encoded a PPO enzyme that is targeted to chloroplasts, whereas PPX2 encoded a mitochondria-targeted PPO enzyme. Given the early use of major PPO inhibitors (e.g., DPEs such as acifluorfen, lactofen, and fomesafen) as primarily foliar-applied herbicides, it was logical to assume that the PPX1-encoded PPO would be the most important of the two target sites, because most of the flux through the protoporphyrin pathway would occur for production of chlorophyll in chloroplasts. Consequently, initial investigations for target-site mutations in the first case of evolved PPO-R, which was in A. tuberculatus, focused on the PPX1 gene (PJT and FED, personal observations). Genetic analysis, however, pointed to PPX2 as the causal resistance gene and led to the first identification of an evolved target-site mutation imparting PPO-R (Patzoldt et al. Reference Patzoldt, Hager, McCormick and Tranel2006).
In hindsight, the realization that a target-site mutation would occur in PPX2 rather than PPX1 makes sense. Watanabe et al. (Reference Watanabe, Che, Iwano, Takayama, Yoshida and Isogai2001) demonstrated that PPX2 from spinach (Spinacia oleracea L.) encodes a dual-targeted PPO enzyme. Specifically, translation of the PPX2-encoded mRNA from the first in-frame start codon resulted in a chloroplast-targeted protein, whereas translation from a second in-frame start codon resulted in a mitochondria-targeted protein. The PPX2 from A. tuberculatus also encodes a predicted chloroplast-targeting sequencing upstream from a second in-frame start codon (Patzoldt et al. Reference Patzoldt, Hager, McCormick and Tranel2006). Consequently, a mutation in PPX2 results in a resistant enzyme in both the chloroplasts and mitochondria. Not all PPX2 homologues in other species are annotated to have an upstream chloroplast-targeting sequence, but dual targeting of the PPX2-encoding enzyme could nonetheless be the norm rather than the exception (Dayan et al. Reference Dayan, Barker and Tranel2018).
It is not clear how important it is that a resistant PPO enzyme be present in both organelles to confer whole-plant resistance. However, of the six weed species identified to date with target-site resistance to PPO inhibitors, five have resistance-conferring mutations in the PPX2 gene and only one has such a mutation in the PPX1 gene. Consequently, the dual-targeting nature of PPO encoded by PPX2 seems to make this gene a more evolutionarily favorable target for providing TSR to PPO inhibitors.
Target-Site Mutations
As mentioned in the preceding section, it was initially surprising when the first target-site mutation for PPO inhibitors was identified in the PPX2 gene. An additional surprise was the type of mutation. Rather than a single-nucleotide polymorphism (SNP) conferring an amino acid substitution (as was the case for all previous target-site mutations), the mutation involved the deletion of three consecutive nucleotides, resulting in a deletion of a glycine residue at position 210, referred to as ΔG210 (Gaines et al. Reference Gaines, Duke, Morran, Rigon, Tranel, Küpper and Dayan2020; Patzoldt et al. Reference Patzoldt, Hager, McCormick and Tranel2006; Figure 6). This codon deletion was fostered by the presence of a small microsatellite repeat (i.e., a bi-repeat of three nucleotides) predisposing the possibility of deletion of a repeat via slippage during DNA replication (Gressel and Levy Reference Gressel and Levy2006). From a weed management standpoint, it is fortunate that the homologous wild-type sequence for the vast majority of weeds do not have such a repeat and, therefore, a homologous ΔG210 mutation is unlikely to evolve in most weeds (Dayan et al. Reference Dayan, Barker and Tranel2018). Palmer amaranth (Amaranthus palmeri S. Watson), however, possesses a repeat homologous to that seen in A. tuberculatus; it was therefore not surprising when the ΔG210 PPX2 mutation was subsequently found in that species (Riggins and Tranel Reference Riggins and Tranel2012; Salas et al. Reference Salas, Burgos, Tranel, Singh, Glasgow, Scott and Nichols2016). To date, however, these are the only two species with this mutation.
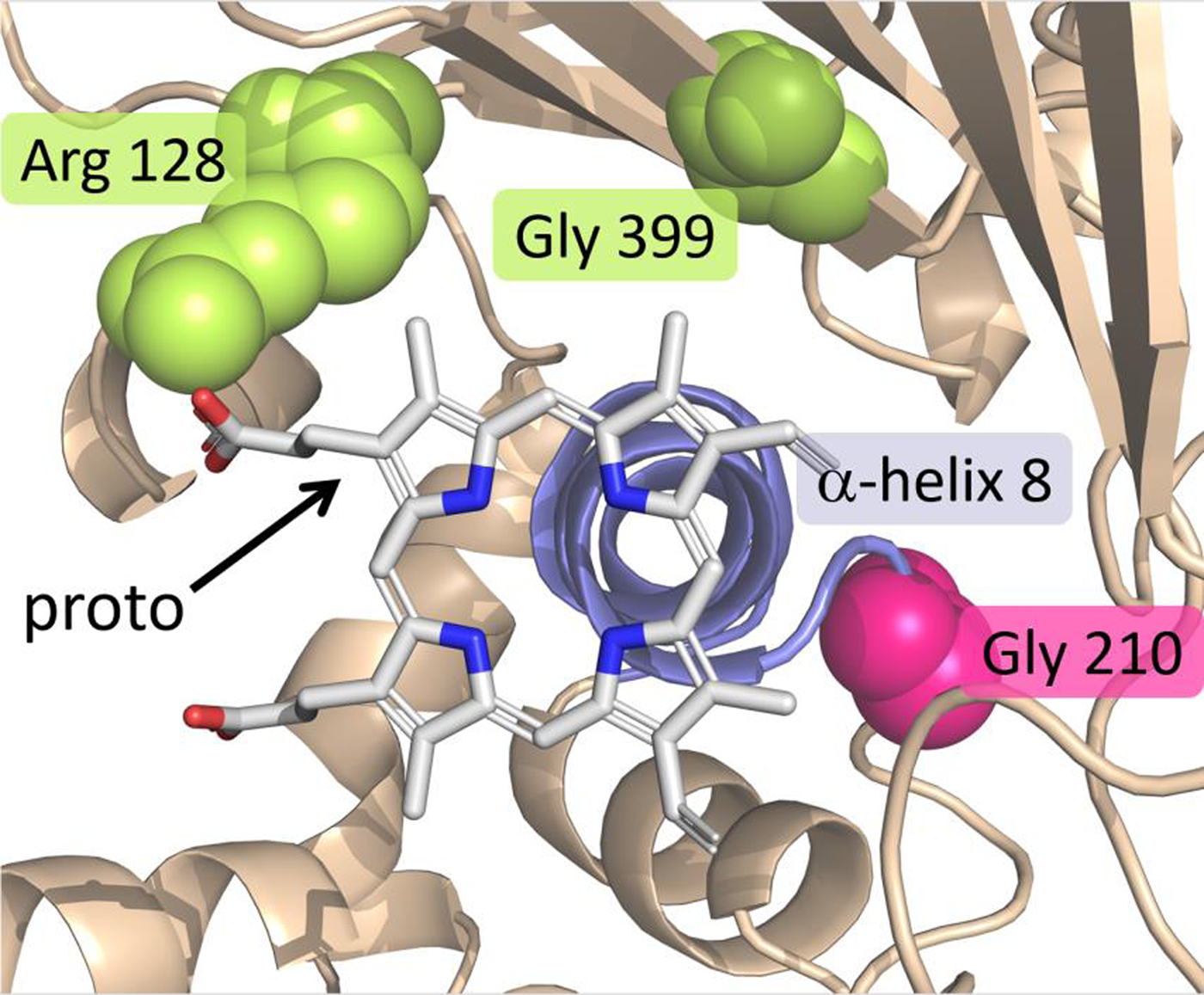
Figure 6. View of the catalytic domain of protoporphyrinogen oxidase (PPO) summarizing the positions where target site–resistance (TSR) mutations can occur. The substrate protoporphyrin is centered on top of α-helix 8 (slate/blue) and stabilized by several interactions with residues lining the pocket. The magenta spheres represent the position of Gly-210, the deletion of which confers TSR. The two groups of yellow/lemon spheres represent Arg-128 and Gly-399, which can be substituted to impart TSR. Adapted from Gaines et al. (Reference Gaines, Duke, Morran, Rigon, Tranel, Küpper and Dayan2020).
The most common mutations conferring TSR to PPO inhibitors result in substitution of an arginine residue at position 128 of the PPX2-encoded PPO. The first such substitution was Arg-128-Leu, identified in common ragweed (Ambrosia artemisiifolia L.) (Table 5). Although initially referred to as Arg-98-Leu, it is now recognized as Arg-128-Leu to be consistent with Gly-210 numbering (Gaines et al. Reference Gaines, Duke, Morran, Rigon, Tranel, Küpper and Dayan2020; Figure 6). To date, a total of four different Arg-128 substitutions have been identified across five weed species. One additional mutation evolved in PPX2 (Gly-399-Ala) confers resistance (Rangani et al. Reference Rangani, Salas-Perez, Aponte, Knapp, Craig, Meitzner, Langaro, Noguera and Burgos2019). To date, this mutation has been observed only in A. palmeri (Gaines et al. Reference Gaines, Duke, Morran, Rigon, Tranel, Küpper and Dayan2020; Figure 6).
Table 5. Chronology of identification in weeds of resistance-conferring Arg-128 substitutions in PPX2-encoded protoporphyrinogen oxidase.

The one incidence of evolved TSR in the PPX1 gene is an Ala-212-Thr substitution, which occurred in goosegrass [Eleusine indica (L.) Gaertn.] (Bi et al. Reference Bi, Wang, Coleman, Porri, Peppers, Patel, Betz, Lerchl and McElroy2020). Although PPX1 and PPX2 are not highly conserved genes, the 212 amino acid position is homologous to the G210 position encoded by PPX2. Interestingly, this mutation was selected by preemergence application of oxadiazon. One would expect the proportional flux through the protoporphyrin pathways in mitochondria versus plastids to be higher in emerging seedlings compared with more established plants. Therefore, it is surprising that the one incidence of a PPX1 mutation occurred due to preemergence selection, whereas the PPX2 mutations are thought to have evolved largely due to postemergence selection. As suggested by Bi et al. (Reference Bi, Wang, Coleman, Porri, Peppers, Patel, Betz, Lerchl and McElroy2020), perhaps different PPO inhibitors have different relative affinities for the two PPO enzymes, with oxadiazon having greater potency on the PPX1-encoded PPO. However, this remains to be determined.
Resistance Magnitude and Cross-Resistance Conferred by Target-Site Mutations
The magnitude of resistance conferred by PPO target-site alterations varies greatly both among alterations and among herbicides. Rangani et al. (Reference Rangani, Salas-Perez, Aponte, Knapp, Craig, Meitzner, Langaro, Noguera and Burgos2019) presented an excellent comparison of resistance levels at the enzyme level, including 13 PPO inhibitors and 3 different PPX2 mutations. In general, both the Gly-399-Ala and ΔG210 alterations conferred broad cross-resistance, with resistant/susceptible (R/S) ratios greater than 100-fold for nearly every PPO-inhibiting herbicide tested. However, the R/S ratio varied across herbicides, in some cases by more than two orders of magnitude. In contrast to Gly-399-Ala and ΔG210 alterations, the Arg-128-Leu substitution generally conferred lower magnitudes of resistance and did not confer any resistance to some PPO inhibitors. Whereas all three of the aforementioned alterations conferred at least some degree of cross-resistance, the Ala-212-Thr PPX1-encoded substitution is more specific, in that it provides a significant level of resistance to oxadiazon only (Bi et al. Reference Bi, Wang, Coleman, Porri, Peppers, Patel, Betz, Lerchl and McElroy2020).
Resistance at the enzyme level does not always translate to the whole-plant level. For example, the Gly-399-Ala substitution conferred greater than 7,000-fold resistance to fomesafen at the enzyme level, but this translated to only a 16-fold resistance in planta (Rangani et al. Reference Rangani, Salas-Perez, Aponte, Knapp, Craig, Meitzner, Langaro, Noguera and Burgos2019). Consequently, even with resistance magnitudes being very high at the enzyme level for many of the alteration-by-herbicide combinations, at the whole-plant level, there is significant variation for resistance at typical field use rates. Therefore, different herbicides will preferentially select for different mutations. For example, in an A. palmeri population segregating for ΔG210, Arg-128-Gly, and Gly-399-Ala alterations, fomesafen survivors collectively contained all three alterations, whereas only plants with the ΔG210 alteration survived saflufenacil (Wu et al. Reference Wu, Goldsmith, Pawlak, Feng, Smith, Navarro and Perez-Jones2020). It is also worth noting that, even with the highest levels of resistance, contact injury typical of PPO inhibitors often is observed after foliar application, but is followed by healthy new growth (Dayan et al. Reference Dayan, Barker and Tranel2018). Such initial injury can make field diagnosis of resistance more challenging.
Coexistence of Different Target-Site Mutations
Among the weeds with TSR, A. palmeri is noteworthy for its high frequencies of different mutations among and within different populations (Noguera et al. Reference Noguera, Rangani, Heiser, Bararpour, Steckel, Betz, Porri, Lerchl, Zimmermann and Nichols2021; Wu et al. Reference Wu, Goldsmith, Pawlak, Feng, Smith, Navarro and Perez-Jones2020). It is the only species to date that has evolved the Gly-399-Ala substitution, the ΔG210 mutation, and two different Arg-128 substitutions. These mutations can coexist within A. palmeri populations, within individual plants, and on rare occasions, even within the same allele (Noguera et al. Reference Noguera, Rangani, Heiser, Bararpour, Steckel, Betz, Porri, Lerchl, Zimmermann and Nichols2021). In contrast, about 95% of the resistance in A. tuberculatus can be attributed to ΔG210 (Nie et al. Reference Nie, Mansfield, Harre, Young, Steppig and Young2019). The high diversity of mutations conferring PPO-R in A. palmeri could be attributed to the relatively high natural tolerance of this species to these herbicides (Lillie et al. Reference Lillie, Giacomini and Tranel2020). Because of its high natural tolerance, even mutations that confer small levels of resistance would be selected. In contrast, in species that have greater inherent sensitivity to PPO inhibitors, such as A. tuberculatus, a more robust mutation (i.e., ΔG210) is needed to confer a selectable level of resistance under typical field conditions. Preferential selection of ΔG210 in A. tuberculatus would be somewhat analogous to the observation made earlier that saflufenacil selected only this mutation in A. palmeri plants segregating for multiple mutations.
Amaranthus PPO2 double mutations of known mutant positions (Gly-399-Ala, Arg-128-Gly, ΔG210, and permutations thereof) on the same allele have strongly reduced in vitro enzyme activity or that many double mutants lead to inactive protein in in vitro PPO2 enzyme assays (Porri et al. Reference Porri, Betz, Seebruck, Knapp, Johnen, Witschel, Aponte, Liebl, Tranel and Lerchl2023). In addition, A. palmeri crossing experiments produced no homozygous ΔG210/Arg-128-Gly double mutations on the same allele in the offspring of several independent crosses (Porri et al. Reference Porri, Noguera, Betz, Sälinger, Brändle, Bowe, Lerchl, Meyer, Knapp and Roma-Burgos2022). These mutations did not segregate according to Mendelian rules. As well, the ΔG210 mutation alone causes a loss of 90% of PPO2 enzyme activity in vitro compared with the unmutated Amaranthus PPO2 enzyme, so there must be a threshold below 10% and above a hence unknown lower activity level that is important for A. palmeri to produce viable offspring. It is still unclear whether A. palmeri homozygous PPX2 double mutants are impaired in seed set or seed viability.
NTSR
As stated previously, NTSR to PPO inhibitors exists in weeds but is poorly characterized relative to TSR. Interestingly, at least some of the cases of NTSR to PPO inhibitors is due to cross-resistance selected by other herbicides (Dimaano and Iwakami Reference Dimaano and Iwakami2021; Mangin et al. Reference Mangin, Hall and Beckie2016; Obenland et al. Reference Obenland, Ma, O’Brien, Lygin and Riechers2019). For example, a population of A. tuberculatus originally identified with metabolic resistance to HPPD inhibitors (Group 27) also exhibited resistance to carfentrazone-ethyl (Obenland et al. Reference Obenland, Ma, O’Brien, Lygin and Riechers2019), even though this PPO-inhibiting herbicide is not widely used for A. tuberculatus control.
As stated earlier, PPO-R is conferred by a variety of target-site mutations in A. palmeri. However, multiple surveys have indicated that NTSR mechanisms (and possibly other as-yet-unknown target-site mutations) could account for resistance in 10% to 25% of resistant A. palmeri plants (Noguera et al. Reference Noguera, Rangani, Heiser, Bararpour, Steckel, Betz, Porri, Lerchl, Zimmermann and Nichols2021; Porri et al. Reference Porri, Noguera, Betz, Sälinger, Brändle, Bowe, Lerchl, Meyer, Knapp and Roma-Burgos2022; Varanasi et al. Reference Varanasi, Brabham, Norsworthy, Nie, Young, Houston, Barber and Scott2018b; Wu et al. Reference Wu, Goldsmith, Pawlak, Feng, Smith, Navarro and Perez-Jones2020). Presumed metabolic resistance in one A. palmeri population was partially reversed by inhibitors of cytochrome P450s and glutathione S-transferases, suggesting the involvement of both of these two enzyme classes (Varanasi et al. Reference Varanasi, Brabham and Norsworthy2018a). This population exhibits not only cross-resistance to multiple PPO inhibitors, but also to Group 2 and 27 herbicides, likely due to NTSR mechanisms (Varanasi et al. Reference Varanasi, Brabham, Korres and Norsworthy2019). Therefore, in this case as well, it is not clear whether PPO-R evolved specifically in response to selection by PPO inhibitors.
Selectivity in Crops
Numerous factors can influence selectivity of herbicides (Blanco et al. Reference Blanco, Ramos, Scarso and de Castro Jorge2015), including chemical and physical properties of the herbicide (such as water solubility, absorbability, lipophilicity, volatility); formulation/adjuvants; placement and metabolic stability in soil; application rate (selectivity window); plant morphology; plant growth stage; application timing; absorption, distribution, metabolism, and excretion characteristics; underlying genetics; and environmental factors (light, temperature, rainfall, humidity, wind). The rapid MoA of PPO-inhibiting herbicides narrows some of these factors. Nonetheless, crop selectivity to Group 14 herbicides involves mostly rapid metabolism of these herbicides (Aizawa and Brown Reference Aizawa, Brown, Böger and Wakabayashi1999; Dayan and Duke Reference Dayan, Duke and Krieger2010), with specific examples for most Group 14 chemical classes, including DPEs (e.g., acifluorfen) (Frear et al. Reference Frear, Swanson and Mansager1983), N-phenyl-imides (e.g., saflufenacil) (Grossmann et al. Reference Grossmann, Hutzler, Caspar, Kwiatkowski and Brommer2011), N-phenyl-triazolinones (e.g., sulfentrazone and carfentrazone) (Dayan et al. Reference Dayan, Duke, Weete and Hancock1997a, Reference Dayan, Weete, Duke and Hancock1997b), and phenylpyrazoles (e.g., pyraflufen-ethyl) (Murata et al. Reference Murata, Yamashita, Kimura, Motoba, Mabuchi and Miura2002). The weed spectrum of PPO-inhibiting herbicides is also strongly mediated by each species’ ability or inability to metabolize these herbicides (Aizawa and Brown Reference Aizawa, Brown, Böger and Wakabayashi1999; Dayan et al. Reference Dayan, Weete and Hancock1996, Reference Dayan, Duke, Weete and Hancock1997a). Although it has been hypothesized (Bi et al. Reference Bi, Wang, Coleman, Porri, Peppers, Patel, Betz, Lerchl and McElroy2020) and is known to exist for ACCase inhibitors (Group 1), target-site selectivity has not been demonstrated for Group 14 PPO-type herbicides in crops.
Most PPO-inhibiting herbicides have strong broadleaf weed activity but are less active on grasses, often due to plant morphology and uptake barriers (i.e., absorption across the cuticle and the cell wall). According to the product labels, this allows the postemergence use of PPO-type herbicides like cinidon-ethyl or flumioxazin in wheat (Triticum aestivum L.) against broadleaf weeds, but it also limits their activity against grasses. Preemergence uses of PPO-inhibiting herbicides like sulfentrazone, saflufenacil, and flumioxazin are strongly dependent on seed placement relative to the position of the herbicide in the soil profile. However, transient injury from preemergence-applied PPO-inhibiting herbicides can happen when the herbicide remains close to the surface and a splashing rainfall event occurs during soybean cracking from the soil that carries the herbicide with the soil particles on the green tissue.
Saflufenacil is a contact burndown herbicide whose preemergence broadleaf weed control in some row crops is dependent on placement (Grossmann et al. Reference Grossmann, Hutzler, Caspar, Kwiatkowski and Brommer2011; Liebl et al. Reference Liebl, Walter, Bowe, Holt and Westberg2008). However, saflufenacil also has some systemic properties involving phloem trapping resulting in injury to meristematic tissues (Grossmann et al. Reference Grossmann, Hutzler, Caspar, Kwiatkowski and Brommer2011). Most other commercial PPO-type herbicides are not systemic, and their translocation is limited by the fast desiccation caused by ROS formation. Addition of ammonium sulfate (AMS) or penetrating adjuvants like methylated seed oils (MSO) can enhance foliar uptake of herbicides, resulting in greater weed control at the expense of a potential loss in crop selectivity and minor injury at labeled use rates (Figure 7). This type of injury does not cause any impact on yield. The structurally related DPE lactofen causes stronger injury and can lead to yield loss.

Figure 7. Transient injury to soybean caused by fomesafen. Symptoms include bronzing and speckled necrosis but rarely lead to long-term impact on yield. Photograph from Maxwel Oliveira, with permission.
Only a few Group 14 herbicides provide sufficient grass control. Pyraflufen-ethyl and carfentrazone can be used postemergence in turfgrass, while oxadiazon and sulfentrazone are used as preemergence herbicides. Again, crop selectivity is largely based on placement, timing, and dose. With the continued emergence of herbicide-resistant grasses throughout agroecosystems around the world, it will be important to design new herbicides with activity on broadleaf weeds and grasses, such as trifludimoxazin, or with very efficient grass control, such as epyrifenacil. These chemistries are not selective for use in aerial spray on crops. Herbicide-tolerance traits would be required for postemergence application in dicot and monocot crops. Hence, the use will be first limited to preemergence applications.
Greenhouse and Lab-screening Methodology for Resistance to PPO-inhibiting Herbicides
Intensive research on PPO-R is likely to continue into the future given recent discovery of novel TSR (Giacomini et al. Reference Giacomini, Umphres, Nie, Mueller, Steckel, Young, Scott and Tranel2017; Rangani et al. Reference Rangani, Salas-Perez, Aponte, Knapp, Craig, Meitzner, Langaro, Noguera and Burgos2019) and NTSR (Varanasi et al. Reference Varanasi, Brabham and Norsworthy2018a) mechanisms and pending commercialization of several new PPO-inhibiting herbicides (e.g., trifludimoxazin, epyrifenacil); therefore, a summary of best practices for PPO-R research is needed. Researchers commonly utilize two methodologies to characterize putative resistant populations: (1) greenhouse dose–response experiments to phenotypically characterize a weed population and (2) genotyping experiments to understand the role of target-site mutations in the phenotypic response. The information herein will describe best practices for these two frequently utilized assays for characterizing PPO-R, focusing primarily on Amaranthus species.
Dose–Response Methods
Dose–response methodology used to characterize herbicide resistance in weeds has been thoroughly documented, most recently by Burgos et al. (Reference Burgos, Tranel, Streibig, Davis, Shaner, Norsworthy and Ritz2013); however, no studies have systematically investigated dose–response methods specific to PPO-R research across the last two decades of literature. For Amaranthus species alone, there have been at least 12 peer-reviewed publications from 2003 to 2020 in which dose–response studies were conducted to characterize PPO-R and PPO-S populations (Table 6) and researchers generated regression estimates (e.g., ED50, LD50, GR50) and subsequently calculated R/S ratios to compare PPO-R and PPO-S populations. Dose–response studies in weed science must include herbicide rates that will generate efficacy values clustered around the key regression estimates for each population. For example, if R/S ratios are calculated from GR50 values, experimental doses should result in multiple data points above and below the 50% value to most accurately predict the GR50 regression estimate. Achieving multiple data points above and below 50% efficacy can be especially challenging in PPO-R Amaranthus research, given that many Amaranthus species are highly sensitive to PPO-inhibiting herbicides applied preemergence or postemergence in greenhouse conditions (Lillie et al. Reference Lillie, Giacomini and Tranel2020).
Table 6. Review of greenhouse dose–response studies on Amaranthus species resistant to protoporphyrinogen oxidase (PPO)-inhibiting herbicides with the most commonly studied PPO-inhibiting preemergence and postemergence herbicides.

a Abbreviations: POST, postemergence (applied after weed emergence); PRE, preemergence (applied before weed emergence).
b The 50% response level was either generated from subjective visual assessments or objective dry biomass relative to a nontreated check.
c Orders of magnitude reflect the span of doses on a single population, not across both populations in cases where staggered rate structures for resistant and susceptible populations were used.
Lactofen and fomesafen were the two most studied herbicides in research investigating PPO-R to postemergence-applied herbicides. Most lactofen studies did not include at least two data points on either side of the 50% efficacy for all populations tested within a study, even if doses spanned three to four orders of magnitude (Table 6). Conversely, studies with fomesafen included proper rates, with doses spanning at least three orders of magnitude or more (Table 6). Achieving full dose–response was challenging in studies of preemergence-applied PPO-inhibiting herbicides. Fomesafen and flumioxazin were the two most tested preemergence-applied herbicides. For flumioxazin, research that utilized a dose range of four to five orders of magnitude and rates as low as 0.001x the recommended dose did not achieve multiple data points above and below 50% efficacy (Schwartz-Lazaro et al. Reference Schwartz-Lazaro, Norsworthy, Scott and Barber2017; Umphres et al. Reference Umphres, Steckel and Mueller2018; Wuerffel et al. Reference Wuerffel, Young, Matthews and Young2015b). This typically occurred because the PPO-S populations were highly sensitive to even the lowest rates tested (0.001x). Dose–response tests using fomesafen were only slightly more successful, with two-thirds of publications including multiple data points above and below 50% efficacy (Table 6).
Molecular Genotyping Methods
Since 2008, when Lee et al. (Reference Lee, Hager and Tranel2008) published the first molecular assay for the detection of the ΔG210 mutation in A. tuberculatus, there have been at least an additional seven publications detailing novel molecular methods for detection of PPO-R in Amaranthus species (Table 7). These molecular methods provide valuable insights on target-site PPO-R at a low cost and/or high throughput. The TaqMan© assay (Applied Biosystems, Thermo Fisher Scientific, Waltham, MA 02451, USA) is the most widely utilized method due to its high-throughput and ability to detect a single PPO-R allele. This assay relies on highly sensitive allele-specific probes to differentiate between PPO-R and PPO-S alleles (Wuerffel et al. Reference Wuerffel, Young, Lee, Tranel, Lightfoot and Young2015a); however, the binding efficiency of these probes can be impacted by minor changes in the targeted sequence (Kaundun et al. Reference Kaundun, Hutchings, Marchegiani, Rauser and Jackson2020). Riggins and Tranel (Reference Riggins and Tranel2012) noted at least six nucleotides within exon 9 of PPX2L on or near the ΔG210 deletion that are present in various Amaranthus species. Given that these nucleotides are present in other species and are not lethal, it is possible that subpopulations of A. tuberculatus or A. palmeri contain these SNPs near the ΔG210 deletion, and it is unclear how these SNPs could impact TaqMan© probe efficiency of either the R or S probe. Other assays, such as the CAPS, dCAPS, and dPACS assays have less throughput than qPCR-based assays (TaqMan© and KASP©, Applied Biosystems, Thermo Fisher Scientific, Waltham, MA 02451, USA) and are also not immune to sources of error.
Table 7. Published genotyping assays for the detection of PPX2L mutations causing resistance to protoporphyrinogen oxidase (PPO)-inhibiting herbicides in Amaranthus species.

a Abbreviations: CAPS, cleaved amplified polymorphic sequence; dCAPS, derived cleaved amplified polymorphic sequence; dPACS, derived polymorphic amplified cleaved sequence; KASP, kompetitive allele-specific PCR.
b EPPO codes: AMATA, Amaranthus tuberculatus; AMAPA, Amaranthus palmeri; AMBEL, Ambrosia artemisiifolia.
How Does Resistance to PPO Inhibitors Compare with Other MoA Resistance?
PPO-inhibiting herbicides have been commercialized for more than 50 years (Appleby Reference Appleby2005), yet weed resistance to these herbicides was not documented until 2001 (Heap Reference Heap2023). Since 2001, PPO-R has been confirmed in 13 other weed species, with a total of 4 species within the Amaranthus genus. Arguably, the most widespread resistance to these herbicides involves A. tuberculatus and A. palmeri, which are prevalent through the U.S. Midwest and Midsouth in soybean and cotton production. The initial lag phase of resistance evolution relative to the period of commercial herbicide use is similar to the pattern observed for resistance evolution to glyphosate or glufosinate and is much longer than the time for weed resistance to evolve to the Group 2 (ALS) and Group 27 (HPPD) herbicides (Heap Reference Heap2023). These differences in the speed of resistance evolution can likely be attributed to the frequency of use and broad adoption of the herbicides, the use of the herbicides in combination with other herbicide MoA groups, the integration of nonchemical weed control practices, and the frequency of robust mutations in weed populations that enable resistance to the specific herbicides.
Another factor that hindered the detection of PPO-R weeds relates to the response of susceptible and resistant A. tuberculatus plants to DPE herbicides. In the 1990s in the U.S. Midwest, DPE herbicides were applied postemergence in soybean production to control A. tuberculatus resistant to the ALS-inhibiting herbicides. The nonsystemic nature of most PPO inhibitors would commonly result in A. tuberculatus survival if the target weed size was larger than ideal or if spray coverage on leaf surfaces was insufficient. Thus, weed survival following application of these herbicides to A. tuberculatus was often put down to adverse application conditions beyond the biological activity limits of the herbicides. Thus, the detection of PPO-R biotypes was likely delayed by several years, as failed weed control was initially attributed to the inconsistency of nonsystemic, foliar application of PPO-inhibiting herbicides.
The complex nature of a codon deletion and the presence of multiple PPO isoforms have contributed to the slow evolution of resistance to PPO inhibitors. As well, other mechanisms imparting resistance to PPO inhibitors include mostly TSR mutations and at least one instance of metabolism-based resistance enabled by enhanced cytochrome P450 and glutathione S-transferase activity in A. palmeri. The spread and evolution of PPO-R has been rampant over the last decade, with double TSR mutations evident with marked frequency in A. palmeri populations in the U.S. Midsouth (Noguera et al. Reference Noguera, Rangani, Heiser, Bararpour, Steckel, Betz, Porri, Lerchl, Zimmermann and Nichols2021). Similar to most forms of herbicide resistance (Cousens Reference Cousens2020), PPO resistance has not been broadly associated with a significant fitness penalty.
The extent of cross-resistance between PPO-inhibiting chemical families varies, depending on the weed species, resistance mechanism, and herbicide application method. In Illinois and Kansas, resistance to postemergence applications of DPE herbicides in A. tuberculatus ranged between 2.2× and 82×, whereas R/S values were less than 6× for the N-phenyl-triazolinone herbicide sulfentrazone and 2.9× to 29× for N-phenyl-imide herbicides (Patzoldt et al. Reference Patzoldt, Tranel and Hager2005; Shoup et al. Reference Shoup, Al-Khatib and Peterson2003; Wuerffel et al. Reference Wuerffel, Young, Matthews and Young2015b). The R/S for fomesafen was reduced from 38× applied postemergence to 3.4× when applied to the soil and evaluating new A. tuberculatus seedling emergence (Wuerffel et al. Reference Wuerffel, Young, Matthews and Young2015b). Similarly, a decline in R/S on PPO-R A. palmeri was observed with fomesafen applied postemergence to 13- to 15-cm-tall (111×) versus 8- to 10-cm-tall (48×) plants, and a further reduction in the R/S occurred when applied preemergence (22.9×) (Lillie et al. Reference Lillie, Giacomini and Tranel2020). In related research, PPO-R A. palmeri populations exhibited variable R/S ratios for fomesafen, flumioxazin, and saflufenacil, with generally lower R/S values when the herbicides were applied preemergence versus postemergence (Schwartz-Lazaro et al. Reference Schwartz-Lazaro, Norsworthy, Scott and Barber2017).
Overall, the numerous TSR mutations, consequent variability in extent and magnitude of cross-resistance, and diversity among weed species with PPO-R is similar to what has been observed in weeds with resistance to Group 1 (ACCase) (Takano et al. Reference Takano, Ovejero, Belchior, Maymone and Dayan2021) and Group 2 (ALS) (Tranel and Wright Reference Tranel and Wright2002) herbicides.
Mutations imparting high-level TSR effectively render these herbicides useless for control of the resistant weed species irrespective of application timing (i.e., preemergence vs. postemergence). However, mutations imparting lower levels of TSR allow these herbicides to retain some commercial value. For example, an A. tuberculatus population with metabolic resistance to atrazine was still controlled by preemergence application of atrazine in field experiments, with an expectation of shorter soil-residual activity than for susceptible populations (Ma et al. Reference Ma, Kaundun, Tranel, Riggins, McGinness, Hager, Hawkes, McIndoe and Riechers2013). Likewise, preemergence applications of isoxaflutole and mesotrione in a field with confirmed A. tuberculatus resistance to HPPD inhibitors resulted in 53% to 90% control at 30 d after treatment (DAT) (Hausman et al. Reference Hausman, Tranel, Riechers, Maxwell, Gonzini and Hager2013). These reports of soil-residual Group 5 (PSII) and Group 27 herbicides maintaining some commercial value for weed populations with documented resistance to the respective herbicide groups are similar to the situation with PPO-R Amaranthus weeds (Wuerffel et al. Reference Wuerffel, Young, Tranel and Young2015c).
Flumioxazin and sulfentrazone are key soil-residual herbicides mentioned by university specialists for Amaranthus spp. control, including those with confirmed PPO-R. The primary drawback is these residual applications still select for higher frequencies of the resistance alleles in A. tuberculatus plants that escape the soil-residual herbicides (Wuerffel et al. Reference Wuerffel, Young, Tranel and Young2015c). Thus, the soil-residual PPO inhibitors should not be applied as the only residual herbicide, and a herbicide from a different MoA group should be the focal herbicide of subsequent postemergence herbicide applications in the field. The fact that PPO inhibitors can still provide commercial value for management of resistant biotypes is the foundation for “next generation” PPO-inhibiting herbicides and crop traits currently in development by private industry as future management tools.
PPO Biochemistry and Protein Structure as Tools for Optimizing New Herbicide Candidates and Resistance Research
Lead optimization of PPO-inhibiting herbicides has historically been achieved through traditional synthesis and greenhouse screens. A game changer occurred in 2004 when the first PPO2 crystal structure became available (Koch et al. Reference Koch, Breithaupt, Kiefersauer, Freigang, Huber and Messerschmidt2004), thereby opening new avenues for biorational design of highly potent PPO-inhibiting herbicides. This structure-based design approach was used for the development of trifludimoxazin (Porri et al. Reference Porri, Betz, Seebruck, Knapp, Johnen, Witschel, Aponte, Liebl, Tranel and Lerchl2023). Trifludimoxazin has novel properties that contribute to a more stable binding compared with other PPO-inhibiting herbicides. One feature is complementary shape between ligand and protein, leading to many favorable van der Waals interactions (Porri et al. Reference Porri, Betz, Seebruck, Knapp, Johnen, Witschel, Aponte, Liebl, Tranel and Lerchl2023). In addition, the molecule is stabilized by pi-stacking effects of the ring systems. The main structural features (Figure 8) that contribute to the high affinity are as follows: the propargyl group anchors in a small hydrophobic pocket of the protein; the triazindionthione head group with the sulfur and the two methyl groups fits very well in the hydrophobic environment; the heterocyclic benzoxazinone exhibits many favorable van der Waals interactions with the beta-sheets shaping the binding site of PPO; a salt bridge to Arg-128 can be established upon binding; and the CF2 group, with its multipolar interactions with the carbonyl oxygens of these beta-sheets, is key for affinity on Amaranthus weeds, as seen in the comparison to the non-fluorinated version (without the CF2 group) (Figure 9). The CF2 group increases the inhibition potency (IC50) of trifludimoxazin compared with the non-fluorinated version as well as lower soil mobility. In addition, the CF2–backbone interactions are not sensitive to the known resistance mutations (Figure 10A). Trifludimoxazin is also a better PPO inhibitor, relative to other benchmark products such as fomesafen and saflufenacil, with lower IC50 values on the wild-type and mutated PPOs (Table 8). The ΔG210 deletion and Arg-128-Gly substitution cause opening of the nearby active site regions, results in a solvation of the binding pocket, while Gly-399-Ala produces electrostatic repulsive interaction with PPO-inhibiting herbicides. While those effects strongly prevent binding of most PPO-inhibiting herbicides to PPO, trifludimoxazin can overcome these target-site mutations through the strong interactions of CF2 with the beta sheet (Figure 10B).

Figure 8. Unique binding properties of trifludimoxazin. The propargyl group (red) anchors at the end of the conserved helix. In the aliphatic region (violet), sulfur fits much better than oxygen. Methyl groups (green) lie in the hydrophobic environment, which enhances binding. The heterocyclic ring (blue) stabilizes binding in protoporphyrinogen oxidase (PPO) compared with other ring structures. The CF2-moiety (pink) provides a strong dipolar interaction.

Figure 9. Comparison of trifludimoxazin (left) properties with its nonfluorinated analogue (right). The fluorinated version shows improved herbicidal activity on Amaranthus spp., improved inhibition potency, and lower mobility in the soil. The K oc value is the partition coefficient of a molecule in a standardized water/n-octanol solvent (logP) normalized to soil organic content. The higher the K oc value, the less mobile the compound is. Fluoro atoms increase lipophilicity and thus increase the K oc value, which equates to lower soil mobility. Hence, less active ingredient can be solved in soil pore water due to adhesion to organic soil matter (Reddy and Locke Reference Reddy and Locke1994).

Figure 10. (A) Binding mode of trifludimoxazin with protoporphyrinogen oxidase (PPO). Affinity to the target is ensured by strong interaction with Phe-381, Gly-382, and Val-383 beta-sheet backbone (white). Trifludimoxazin is shown in magenta sticks, and heteroatoms are colored: oxygen in red, nitrogen in blue, sulfur in yellow, and fluorine in green. (B) Binding mode of trifludimoxazin to PPO with the target-site mutations Arg-128-Gly, G398A, and ΔG210 (labeled). The shape of the binding pocket of PPO wild type is depicted by white meshes. Wild-type residues G210 and R128 are shown in green. The shape of the Arg-128-Gly + G398A double mutant is depicted by cyan meshes, the corresponding residues are colored cyan. G398A will push PPO inhibitors away from the beta-sheet structures. This worsens the fit between PPO and inhibitor and, consequently, the affinity. In the case of the mutation R128A, inhibitors may lose a salt bridge, and the shape of the binding site is much shallower, such that water molecules can easily access the site and compete with inhibitor binding. This is also true for the deletion of G210. More easily accessible binding pockets of PPO mutants are indicated by blue arrows.
Table 8. Inhibition of protoporphyrinogen oxidase (PPO) enzyme activity in wild-type AMATU PPO1 and PPO2, as well as PPO2 target-site mutants, heterologously expressed in Escherichia coli.

Trifludimoxazin had very good efficacy against PPO-resistant Amaranthus biotypes possessing the G210 deletion (Figure 11); 12 g ha−1 and 18 g ha−1 of trifludimoxazin resulted in 88% and 96% control of an Amaranthus ΔG210 biotype, respectively, while saflufenacil and lactofen at 50 g ha−1 and 420 g ha−1, respectively, provided less than 50% control. These results suggest that trifludimoxazin can be used to control PPO-resistant biotypes with currently known target-site mutations (Porri et al. Reference Porri, Betz, Seebruck, Knapp, Johnen, Witschel, Aponte, Liebl, Tranel and Lerchl2023).

Figure 11. Efficacy of trifludimoxazin against ΔG210 Amaranthus turberculatus mutants under field conditions. Percent control 15 d after treatment (DAT). Treatments included 1% methylated seed oil (MSO) and 1.2% (AMS) (1.68 kg ha−1).
As suggested, trifludimoxazin is effective against naturally occurring PPO2 double target-site mutations, such as ΔG210 R128X (“X” indicating any possible amino acid) at the enzyme level (Porri et al. Reference Porri, Betz, Seebruck, Knapp, Johnen, Witschel, Aponte, Liebl, Tranel and Lerchl2023). The IC50 values of trifludimoxazin toward ΔG210 R128X are much lower than the fomesafen, lactofen, and saflufenacil values, indicating a superior inhibition potency of trifludimoxazin against PPO double target-site mutations (Porri et al. Reference Porri, Betz, Seebruck, Knapp, Johnen, Witschel, Aponte, Liebl, Tranel and Lerchl2023). Some ΔG210 R128X enzyme variants, including ΔG210 Arg-128-Gly are inactive as confirmed at the plant level, as Amaranthus plants carrying ΔG210 Arg-128-Gly homozygous are impacted in seed biology (Porri et al. Reference Porri, Noguera, Betz, Sälinger, Brändle, Bowe, Lerchl, Meyer, Knapp and Roma-Burgos2022). Other ΔG210 R128X mutations may occur, but protein activity is strongly reduced, such that a fitness cost could be expected, making the occurrence of these double mutations less likely (Lerchl et al. Reference Lerchl, Hedtke, Wittmann, Noguera, Porri, Meyer, De, Tranel, Seebruck, Roma-Burgos and Grimm2023). Triple target-site mutations can be expected to affect protein activity more strongly, hence knocking out protein function.
As reported previously, artificially engineered combinations of double or triple PPO2 mutations can lead to a robust herbicide tolerance toward trifludimoxazin when expressed in key crops (Aponte et al. Reference Aponte, Mankin, Tresch, Lerchl, Witschel, Mietzner, Armel and Liebl2017). However, these mutations are highly unlikely to occur through mutations in weeds. Other not yet reported natural PPO target-site mutations may appear in the future and could potentially reduce the affinity of trifludimoxazin toward the PPO enzyme, especially if more than one amino acid substitution occurs, rendering the enzyme resistant with protein activity above the weed´s threshold for growth and development. However, trifludimoxazin binding relies heavily on the interaction between the CF2 group and the beta sheet of the PPO protein, a side chain region that is less prone to sequence variation. Hence, mutations in this region are less likely to occur.
Resistance Management Principles for Sustainable Weed Management: A Plan of Action
The elimination of all herbicides in a weed management strategy would be the only sure method to stop the evolution and spread of weed resistance to herbicides. However, the numerous benefits that these tools bring to farmers, and society as a whole, have kept them at the forefront of weed management practices. Furthermore, the evolution of resistance to nonchemical weed control can be fast. In lieu of eliminating herbicide use, mitigating further evolution or spread of herbicide resistance is paramount and will require a holistic approach of cooperation among farmers, Extension agents, farm advisors, scientists, industry, and regulators (Table 9).
Table 9. Examples of recommendations for management with protoporphyrinogen oxidase (PPO) inhibitors.

Sustainable Programs Built upon Science
The importance of a diversified weed management program that focuses on reducing the weed seedbank through planting into fields free of weeds, rotating crops, maximizing crop competitiveness, integrating nonchemical practices, removing weed escapes, and using herbicide programs focused on diversity of chemistry within and across years has been promoted for decades (Beckie Reference Beckie2006; Walsh and Powles Reference Walsh and Powles2007). Currently, weed scientists are actively studying how cover crops, deep tillage, in-row cultivation, varying row spacings, hand weeding, and weed seed destruction at harvest can be used to lessen herbicide selection pressure (Bell et al. Reference Bell, Norsworthy and Scott2016; Bunchek et al. Reference Bunchek, Wallace, Curran, Mortensen, VanGessel and Scott2020; Hay et al. Reference Hay, Dille and Peterson2019; Price et al. Reference Price, Balkcom, Duzy and Kelton2012; Walsh et al. Reference Walsh, Harrington and Powles2012; Wiggins et al. Reference Wiggins, Hayes and Steckel2016). For example, a cereal rye (Secale cereale L.) cover crop reduced selection pressure to postemergence herbicides on A. palmeri in a cotton system by 77% during the early season and by 65% for the entire season (Hand et al. Reference Hand, Randell, Nichols, Steckel, Basinger and Culpepper2021). This cover crop also reduced the number of plants present after the first postemergence application by 43%, thereby further reducing herbicide selection pressure from a second postemergence application.
Although nonchemical control tactics are a critical component of any sustainable management system, herbicides remain the strongest and most economical approach to manage weeds in large-scale agriculture (Gianessi and Reigner Reference Gianessi and Reigner2007; Hay et al. Reference Hay, Dille and Peterson2019). Thus, scientists work diligently to maximize herbicide MoA diversity and agricultural practices within agronomic systems. These include life cycle management and innovative tank mixes of existing herbicide chemistries, overlapping residual herbicides with multiple MoAs through the season, improving application timings, understanding ideal environmental conditions for herbicide activation, and improving on-target applications, all of which are critical to resistance management programs (Osterholt et al. Reference Osterholt, Webster, Blouin and McKnight2019; Powles et al. Reference Powles, Preston, Bryan and Jutsum1996; Sarangi and Jhala Reference Sarangi and Jhala2019; Stewart et al. Reference Stewart, Nurse, Hamill and Sikkema2010; Walsh and Powles Reference Walsh and Powles2007).
While scientists must understand the resistance mechanism to more effectively deploy field management recommendations (Gressel Reference Gressel2009), we must get past the unsustainable management practice of adopting a new herbicide MoA to control a weed resistant to an older herbicide (Duke and Dayan Reference Duke and Dayan2022). In some instances, management of herbicide-resistant weed biotypes may involve the continued use of that same herbicide MoA, but with application methods that can at least partially minimize the impact the resistance mechanism has on weed management in the field (Wuerffel et al. Reference Wuerffel, Young, Tranel and Young2015c).
Designing an effective herbicide program as part of a holistic system to manage resistant weeds at the farm level also requires an understanding of how each herbicide within an MoA group performs. PPO-inhibiting herbicides are an ideal example of this challenge. Foliar applications of PPO inhibitors on resistant populations of Amaranthus most often fail (Copeland et al. Reference Copeland, Giacomini, Tranel, Montgomery and Steckel2018; Lillie et al. Reference Lillie, Giacomini and Tranel2020). Conversely, soil-residual applications of fomesafen, flumioxazin, and sulfentrazone control most PPO-resistant populations, albeit with a shorter length of residual control while shifting toward a greater frequency of the resistance alleles in any surviving weeds (Lillie et al. Reference Lillie, Giacomini and Tranel2020).
The U.S. Environmental Protection Agency (USEPA), U.S. Department of Agriculture (USDA), and State Lead Pesticide Agencies Must Contribute to Diverse Programs
Farmers need enhanced cooperative efforts among weed scientists, USDA, USEPA, and state lead agencies working jointly to address resistance challenges. The USDA plays a critical and direct role that could change the scope of herbicide resistance immediately by fostering herbicide stewardship, using sound science and common sense, analogous to how it fostered the adoption of conservation tillage programs. If farmers have access to state and federal funds to conserve soil, why would funding designated for herbicide stewardship, which can reduce the need for tillage to control weeds, be unrealistic? Perhaps a tiered incentive program can reward farmers for implementing weed management strategies that integrate one or more practices that are nonchemical, or at least demonstrate control of the majority of the weed population does not rely on any single herbicide active ingredient or MoA group. Incentive programs designed to reduce the number of weeds by chemical control while improving soil health, production, and the carbon footprint would be well received (Schroeder et al. Reference Schroeder, Barrett, Shaw, Asmus, Coble, Ervin, Jussaume, Owen, Burke and Creech2018).
The USEPA’s ability to influence herbicide availability through registration or cancellation of registration, as well as its influence on label requirements and recommendations, empowers it to arguably be the most influential single entity impacting a farmer’s ability to implement sound resistance management programs in the United States. Being knowledgeable about weed resistance to herbicides and its impact on agriculture (USEPA 2017), the USEPA needs to take a more active role in facilitating practical and economical solutions to address this threat. The agency has recognized the importance and need for methods to drive the development of new herbicides through regulatory incentives (Barrett et al. Reference Barrett, Barrett, Soteres and Shaw2017), and a process that would lessen the cost and amount of time required for registrations would empower more competition, thereby likely developing more tools available for farmers. Additionally, the USEPA can help farmers immediately by creating incentives for registrants to overhaul current archaic labeling protocols. A recent survey noted 45% of farmers felt herbicide label directions were not overly effective in curbing the development of herbicide resistance (Steckel Reference Steckel2018). Federal labels developed with a one-size-fits-all approach should be rapidly replaced with more targeted labels providing regional crop-specific and, in time, more locally developed recommendations. The theory that a resistance management program for Amaranthus using a given herbicide in soybean grown in the Midwest should be similar to the use of that same product in cotton growing in the Southeast is flawed. Management programs developed and shared at the local level will foster greater adoption of sustainable programs, use patterns, rates, and so on with improved pesticide stewardship (Culpepper et al. Reference Culpepper, Vance, Gray, Johnson and Prostko2020). Additionally, this process would allow more accurate documentation of overall pesticide use, directly influencing risk cup and pesticide use calculations/modeling. The risk cup is the USEPA’s conceptual approach to estimating total pesticide exposure and risk according to the Food Quality Protection Act (USEPA 1996). The agency must also evaluate how in-field buffer regulation, county-wide use restrictions, and cutoff application dates remove the use of herbicides on large land areas, thereby facilitating rapid resistance development to the limited number of other management options.
State lead pesticide agencies are often overlooked important partners in developing resistance management plans. The ability of states to develop recommendations and labels (e.g., FIFRA Section 24(c), Section 2(ee), and Section 18 labels) places them in a unique position to foster label development from locally generated science and states should be a partner in developing regional or local smart labels. Additionally, these agencies influence training materials that pesticide applicators are required to understand to obtain an applicator’s license and to apply certain pesticides; herbicide-resistance evolution and management education should be a part of these training efforts.
Industry Must Incentivize Alternative Weed Control Options Reducing Selection Pressure to Their Products
While the agricultural industry continues to offer critical chemical solutions in weed control, Harr (Reference Harr1992, p. 177) recognized three decades ago that “as cropping systems and criteria for desirable weed control levels change, industry will have to change from a reactive to a proactive participant in the development of integrated solutions.” He also noted that “industry will not only have to offer those services but at the same time assist in the education of growers to enable efficient use of the increasingly intricate methods of future weed control.” These concepts have never been more relevant, and the need for industry to work with academics, economists, regulators, and farmers to improve weed management programming has reached a critical point. Working with regulators and academics to develop proactive diversified programs will foster the sustainability of farms in those geographies where this cooperation occurs. Most importantly, industry must reevaluate its farm-based incentive program approaches with the priority of ensuring resistance management programs are implemented, even if that includes utilizing competitors’ products. Nevertheless, it should be recognized that in recent years, HRAC has partnered with the Weed Science Society of America (WSSA) to update the herbicide MoA classification and generate one global classification. Through partnership with CropLife International, herbicide labels now clearly mention the MoA of each compound. In addition, efforts have been made to improve the communication on integrated weed management with all stakeholders (HRAC 2022), including the generation of herbicide use recommendations (e.g., for Group 27, HPPD inhibitors; and Group 15, VLCFA inhibitors).
Farmers, Extension, and Advisors Must Make Responsible Decisions
Farmers overwhelmingly favor low-cost weed management to maximize profits, a strategy that readily translates to herbicides only, and as few herbicides as possible, to kill weeds (Schroeder et al. Reference Schroeder, Barrett, Shaw, Asmus, Coble, Ervin, Jussaume, Owen, Burke and Creech2018). The inevitable path toward selection of herbicide resistance will only be redirected if the economics of herbicide stewardship change. Thus, an incentivized approach coordinating educational programs between federal and state lead agencies, Extension, registrants, and retailers would immediately bear fruit, with farmers implementing more diversified weed management programs.
Exploiting the weeds’ biological weaknesses (Bhowmik Reference Bhowmik1997) and incorporating cover crops or tillage to manage seed emergence, seed viability, and weed competition for specific local needs would have the greatest influence on farmers’ decisions. Planting weed-free crop seed, scouting routinely, implementing timely herbicide application, ideal nutrient and irrigation management, minimizing spread of seeds or propagules, and rotating crops when economically feasible are also important responsibilities the farmer must take seriously in order to achieve successful weed management (Burgos et al. Reference Burgos, Norman, Gealy and Black2006; Gonzalez-Andujar et al. Reference Gonzalez-Andujar, Plant and Fernandez-Quintanilla2001; Neve et al. Reference Neve, Norsworthy, Smith and Zelaya2011).
The WSSA Needs to Foster Enhanced Cooperation among Scientists, Regulators, Advisors, and Farmers
The WSSA promotes research, education, and Extension outreach activities related to weeds; provides science-based information to the public and policy makers; fosters awareness of weeds and their impact on managed and natural ecosystems; and promotes cooperation among weed science organizations across the nation and around the world (WSSA 2022). Without question, a long-term sustainable solution for implementing resistance management strategies on the farm begins with cooperation among the critical parties. The WSSA holds the unique capacity for and challenge of fostering relationships among these entities, facilitating approaches that can lead to methods that assist in the sustainability of our farmers as they work tirelessly to provide food, feed, and fiber for the world.
Acknowledgments
This work was partially funded by USDA Cooperative Agreement 58-6060-6-015 grant to the University of Mississippi (SOD) and USDA National Institute of Food and Agriculture, Hatch Projects 1016591/COL00785 (FED) and ILLU-802-944 (PJT). No conflicts of interest have been declared.