(Poly)phenols are naturally occurring secondary metabolites ubiquitous across a wide range of plant foods and beverages such as tea, coffee, wine, chocolate, berries, citrus, nuts, soy and some vegetables. Large-scale epidemiological studies have estimated that the total (poly)phenol intake in Europe ranges between 584 and 1786 mg/d(Reference Wisnuwardani, De Henauw and Androutsos1,Reference Zamora-Ros, Knaze and Rothwell2) . They exist as a substantial variety of compounds with diverse structures, and they are broadly classified into 2 main groups: flavonoids and non-flavonoids. Among the flavonoids, 6 main subclasses exist: anthocyanins, flavan-3-ols, flavonols, isoflavones, flavanones and flavones (Fig. 1). Among non-flavonoids, some of the major subclasses include phenolic acids, lignans, stilbenes, tannins and coumarins. In plants, these phytochemicals serve many roles including colouration for pollination, shielding from UV-B radiation and protection against natural pathogens and herbivores(Reference Alappat and Alappat3–Reference Singh, Kaur and Kariyat5). This sparked interest in understanding and exploiting their health benefits. In recent decades, there have been considerable advances in (poly)phenol research, particularly in the context of human health. Therefore, this review will attempt to provide a summary of the current state of the art and future trends related to these food bioactives. Current evidence derived from epidemiological and clinical studies will be presented along with their hurdles and limitations. To better elucidate the causal link, the review will also touch on the molecular mechanisms pertaining to the observed effects followed by the tight-knit relationships between (poly)phenols and the gut microbiota.
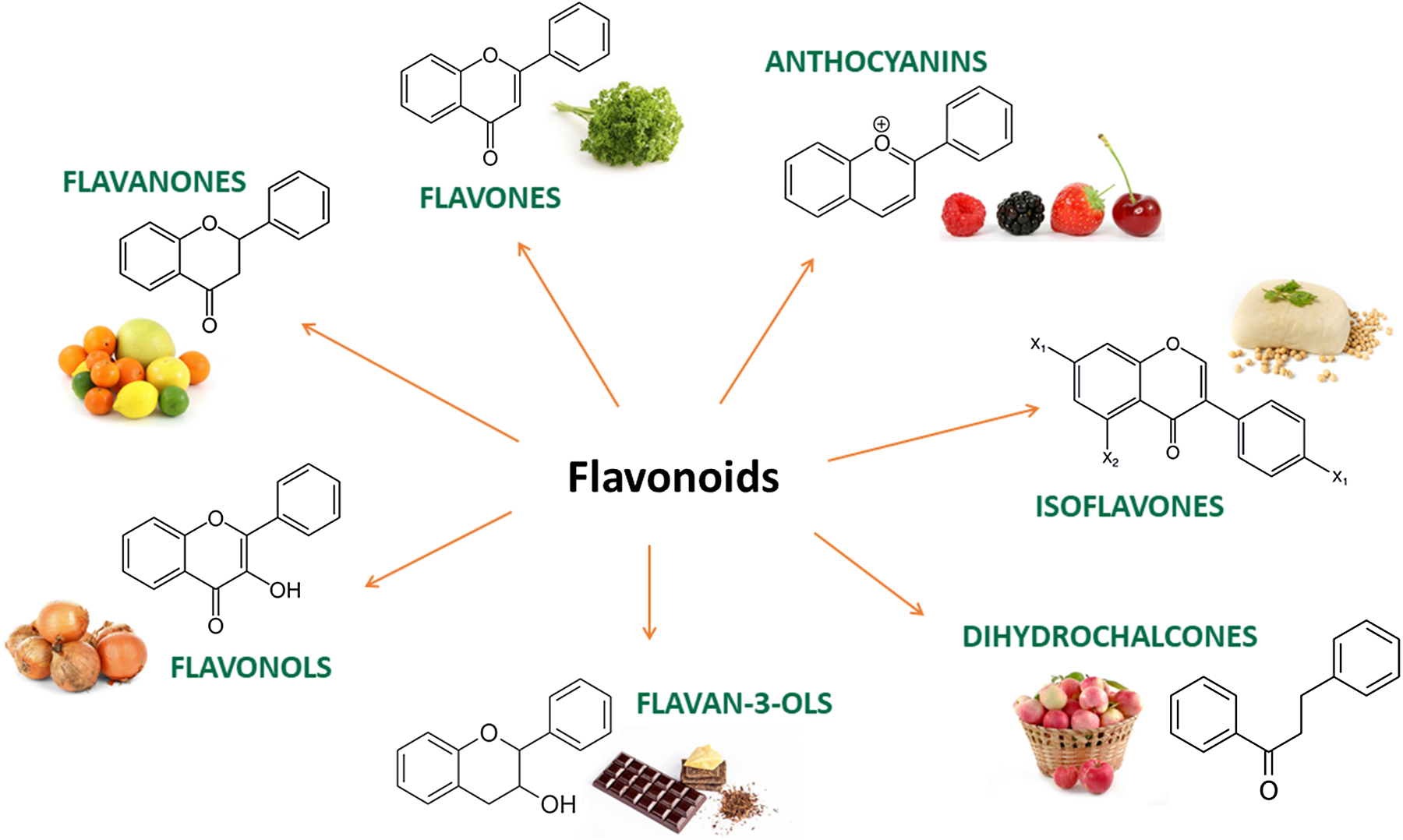
Fig. 1. Main subclasses of flavonoids and examples of food sources.
Effects of (poly)phenols on cardiometabolic health: epidemiological evidence
Currently, a significant number of meta-analyses focusing on observational studies investigating associations between (poly)phenols and health outcomes have been published. In the context of cardiometabolic health, data for the subclass of flavonoids is particularly strong. In one of the most comprehensive approaches, a meta-analysis of 39 prospective cohort studies comprising over 1·5 million individuals suggested that an intake of up to 500 mg/d of total flavonoids was associated with a 27 %, 20 % and 23 % lower relative risk (RR) of cardiovascular disease (CVD), stroke and coronary heart disease (CHD), respectively(Reference Micek, Godos and Del Rio6). This improvement can mainly be attributed to its subgroups – anthocyanins and flavan-3-ols showing an inverse association with CVD risk, while the flavonols and flavones were linked to reduced CHD risk. Flavanone intake was associated with reduced risk of stroke in a linear inverse manner, whereas flavan-3-ol monomers showed positive associations towards all cardiovascular outcomes. As a whole, higher intakes were associated with 9 %–25 % lower risk of CVD, 2 %–30 % lower risk of stroke and 1 %–21 % lower risk of CHD across all the subclasses of flavonoids. In another review worth noting, the meta-analysis consolidated findings across 15 cohorts (23 publications) evaluating the impact of flavan-3-ols intake on cardiometabolic outcomes(Reference Raman, Avendano and Chen7). Overall, higher intake of total flavan-3-ols was associated with 13 % lower risk of CVD, 19 % lower risk of CHD, 5 % lower risk of stroke and 32 % lower risk of type-2 diabetes mellitus (T2DM). As for proanthocyanidins specifically, only the association between higher intakes and 11 % lower T2DM risk was statistically significant (RR = 0·89, 95 % CI: 0·81, 0·98).
Although other (poly)phenols such as phenolic acids, lignans and stilbenes have been picking up considerable interest as of late, large-scale observational studies specific to them remain scarce, let alone their corresponding meta-analyses. Phenolic acids are simpler forms of dietary (poly)phenols ubiquitous across most diets and major food sources of (poly)phenols. Intake of phenolic acids ranged widely across different regions and populations from 100 to 800 mg/d(Reference Del Bo, Bernardi and Marino8) with coffee being the main food source estimated from the European Prospective Investigation into Cancer and Nutrition (EPIC) cohort(Reference Zamora-Ros, Rothwell and Scalbert9). Comprehensive meta-analysis linking phenolic acids to cardiometabolic health is currently lacking, but individual observational studies have reported that high intake was associated with lower odds of metabolic syndrome and elevated systolic blood pressure (SBP)(Reference Lanuza, Zamora-Ros and Bondonno10), CVD(Reference María Mérida, Vitelli-Storelli and Moreno-Franco11,Reference Vázquez-Ruiz, Toledo and Vitelli-Storelli12) , non-alcohol fatty liver disease (NAFLD) and insulin resistance(Reference Salomone, Ivancovsky-Wajcman and Fliss-Isakov13). As for lignans, the sub-analysis from the aforementioned meta-analysis(Reference Micek, Godos and Del Rio6) estimated that when compared between the highest v. lowest group, intake was associated with 18 % lower risk of CVD, 8 % lower risk of stroke and 18 % lower risk of CHD. This echoed well with a smaller but noteworthy meta-analysis looking specifically at lignan intake and cardiometabolic outcomes(Reference Xia, Chen and Li14). Across 12 prospective cohort studies, they suggested that the highest intake of lignans was correlated with 15 % reduced risk of CVD and T2DM. Their dose-dependent analysis revealed that every 500 μg/d increment translated to RR of 0·83 (95 % CI: 0·74, 0·92) for CVD and 0·96 (95 % CI: 0·95, 0·98) for T2DM. Today, meta-analyses specific to the stilbene subclass as a whole remain a paucity while research on the individual stilbene, resveratrol was mainly centred around clinical work.
Though findings derived from epidemiological studies are promising, a number of limitations need to be considered. Estimating dietary intake of (poly)phenols has always been challenging. To begin with, our recent systematic review aimed at characterising methods used by observational studies found that a staggering 74 % of the studies failed to employ validated methods for appropriate measures of the target (poly)phenols(Reference Xu, Le Sayec and Roberts15). The review also revealed that food frequency questionnaires (FFQ) were still by far the most common assessment tool for dietary (poly)phenol intake, likely due to their relatively low burden towards participants and researchers. FFQs are not an optimal tool for estimating intake due to the limited range of food sources listed in the questionnaires, especially for (poly)phenol food sources, which are not well represented. For instance, FFQs typically cannot distinguish between red and white wine, or between different types of tea and coffee, which contain significantly different polyphenol content. Berries are often not reported, as well as nuts, herbs and spices. Although regional FFQs do exist, the predefined list of food groups and items in FFQs are likely developed according to Western or European diets, limiting their adaptability and extrapolability to other countries. As an example, foods such as phytochemical-rich tropical fruits and vegetables, although exotic to the West, are ubiquitous in the habitual diets of populations in some regions. Ideally, FFQs designed for specific regions or population groups should be used. This is easier said than done judging from the fact that accurate large-scale national-level datasets would be required to develop an effective FFQ to start with, let alone having it validated for specialised use cases such as (poly)phenol assessment. Another important limitation related to (poly)phenol intake estimation is the current state of (poly)phenol composition databases since the ones available are considerably lacking many important foods. Besides individual food components, modern diets and food preparation techniques inevitably create complicated food matrices, further attaching an additional layer of complexity to dietary assessment. Some have proposed not to focus solely on individual foods or food components but rather on the nutritional characteristics of meals, dishes and diets, as they are actually consumed(Reference Marconi, Durazzo and Camilli16). Direct chemical analysis of foods would be the most accurate, but it is not always practical, especially for long-term studies. With the current ordeal of incomplete food databases together with the high variability of reported (poly)phenol content in foods(Reference Manach, Scalbert and Morand17) coupled with the added complexity of modern dietary habits, the over- and under-reporting of intake has become commonplace due to unavoidable guesswork. As it currently stands, evidence supporting the use of traditional self-reported assessments is reasonably weak(Reference Ottaviani, Schroeter and Kuhnle18) and better strategies are needed. This eventually led to the recent paradigm shift towards biomarkers as surrogates for intake and dietary exposure. The biomarker approach requires analytical evaluation of (poly)phenol metabolites in biofluids to essentially reverse-trace a (poly)phenol from its end-stage metabolites towards its precursor and subsequently to its sources in food(Reference Scalbert, Brennan and Manach19). Currently, this tool has been applied only in a few observational studies. A cross-sectional analysis of the EPIC Norfolk cohort comprising over 25 thousand participants revealed that biomarker-estimated high flavan-3-ol intake was associated with significantly lower systolic blood pressure at –1·9 (95 % CI: –2·7, –1·1) mmHg in men and –2·5 (95 % CI: –3·3, –1·8) mmHg in women(Reference Ottaviani, Britten and Lucarelli20). Importantly, no correlations were found between biomarkers and flavan-3-ol intake estimated using 7-d food diaries. This is in line with recent work from our group showing that a large panel of (poly)phenol metabolites were poorly correlated with estimated intake from FFQs and 7-d food diaries(Reference Xu, Li and Ma21). A small meta-analysis of eight observational studies found that higher enterolactone (a metabolite of lignans) concentration was associated with a 30 % and 45 % reduced all-cause and CVD mortality risk(Reference Rienks, Barbaresko and Nöthlings22). Though promising, it is important to discuss the shortfall of using biomarkers of (poly)phenol intake as a tool, which needs careful evaluation and validation. To our knowledge, no biomarker of (poly)phenol intake has been fully validated to date. One of the major obstacles to developing biomarkers of intake for (poly)phenols seems to be the high inter-individual variability in absorption and metabolism reported, in particular for gut microbial metabolism(Reference Landberg, Manach and Kerckhof23). On top of that, the interactions between (poly)phenols and other food components further complicate this. For example, recent studies have shown how (poly)phenol oxidase-rich foods such as bananas dramatically influenced the bioavailability of flavan-3-ols when consumed together(Reference Ottaviani, Ensunsa and Fong24), or the increase in bioavailability and efficacy of flavan-3-ols when methylxanthines were present(Reference Ottaviani, Fong and Borges25,Reference Sansone, Ottaviani and Rodriguez-Mateos26) . Another issue is the lack of specificity of certain (poly)phenol metabolites. For example, the main metabolites of anthocyanins include a combination of derivatives of cinnamic acids, benzoic acids, catechols, pyrogallols and hippuric acids, which are also metabolites derived from most (poly)phenol subclasses and from endogenous metabolism. A plausible solution to this is to resort to multi-metabolite panels consisting of several metabolites to use as biomarkers of intake. Such an approach has been exploited in initial efforts to discover biomarker candidates for foods such as berries and grapes(Reference Mostafa, Cheok and Meroño27,Reference Ulaszewska, Garcia-Aloy and Vázquez-Manjarrez28) , and also for flavan-3-ols(Reference Ottaviani, Fong and Kimball29,Reference Ottaviani, Fong and Kimball30) . At the current state, this multi-metabolite strategy, although not perfect, remains a good alternative to dietary assessment methods to estimate exposure and intake.
Effects of (poly)phenols on cardiometabolic health: clinical evidence
Large-scale epidemiological studies are generally effective in unearthing potential associations between diet and disease outcomes. However, they struggle when it comes to identifying causal links. Randomised controlled trials (RCTs) for many (poly)phenols do exist today, followed by their corresponding meta-analyses. Among all (poly)phenols, clinical work around flavan-3-ols is currently the most comprehensive. These findings were summarised in a recent meta-analysis(Reference Raman, Avendano and Chen7) looking at the effects of flavan-3-ols intake on cardiometabolic risk factors which included 157 RCTs. The flavan-3-ols interventions were administered in the form of purified compounds, food extracts, fruits, cocoa, teas and wines with dosages ranging from 40–1560 mg/d over periods of 3–26 weeks. Overall, flavan-3-ol supplementation resulted in significant improvements in total cholesterol (TC), LDL and HDL cholesterol (LDL-C and HDL-C), triglycerides (TG), diastolic blood pressure (DBP), haemoglobin A1c (HbA1c), homeostasis model assessment of insulin resistance (HOMA-IR) and in both acute and chronic flow-mediated dilation (FMD). When limited to only studies with good methodological quality, all outcomes remained statistically significant except for LDL-C, HbA1c and TG. In 2022, the largest, longest and most robust RCT on (poly)phenols to date was published(Reference Sesso, Manson and Aragaki31). The COcoa Supplement and Multivitamin Outcomes Study (COSMOS) was a large-scale double-blind, 2-by-2 factorial RCT aimed at investigating the impact of cocoa extract and multivitamins supplementation on the prevention of CVD and cancer involving over 21 thousand US adults. Participants were randomised into taking a cocoa extract supplement [500 mg flavan-3-ols/d, including 80 mg (–)-epicatechin] or placebo over a treatment period of 5 years. Over a median follow-up of 3·6 years, 866 participants taking cocoa extract (n 410) and placebo (n 456) had confirmed total cardiovascular events (primary outcome) with hazard rates (HR) of 0·90 (95 % CI: 0·78, 1·02, P = 0·11). When restricted to per-protocol analyses, however, the primary outcome became statistically significant (HR: 0·85; 95 % CI: 0·72, 0·99). Secondary outcomes included MI, stroke, coronary revascularisation, all-cause mortality and CVD death. A 27 % reduced risk of death from CVD was found in the flavan-3-ol group compared with the placebo, while the other secondary outcomes were NS. Such findings are the first suggestive evidence that long-term and continuous supplementation with cocoa flavan-3-ols reduces clinical CVD outcomes. COSMOS has shown that conducting large dietary interventions with hard clinical outcomes is possible, setting a precedent for future efforts.
Compared to flavan-3-ols, clinical research on other (poly)phenols currently is not as extensive but still, there is an increasing number of meta-analyses of RCTs available for most (poly)phenol subclasses. Several meta-analyses have collectively suggested that anthocyanins and anthocyanin-rich foods are able to improve BP, TC, TG, LDL-C, HDL-C, fasting glucose, HbA1c, FMD and pulse-wave velocity(Reference Fairlie-Jones, Davison and Fromentin32–Reference Yang, Ling and Du37). Our group have shown that berry anthocyanins can improve endothelial function in a dose-dependent manner, both as purified anthocyanins and as part of whole berries(Reference Rodriguez-Mateos, Istas and Boschek38,Reference Rodriguez-Mateos, Rendeiro and Bergillos-Meca39) . The lowest amount tested (100 g of fresh blueberries equiv., containing 129 mg of anthocyanins) showed significant improvements in FMD, with a maximum amount equivalent to 240 g of fresh blueberries, after which the effects plateaued and didn’t improve further(Reference Rodriguez-Mateos, Rendeiro and Bergillos-Meca39). A similar dose response was also shown with cranberry (poly)phenols(Reference Rodriguez-Mateos, Feliciano and Boeres40). This is in line with a recent meta-analysis exploring the dose-dependent relationship between cocoa flavan-3-ols and endothelial function(Reference Sun, Zimmermann and De Castro41). They suggested that optimal FMD improvements were observed around 710 mg of total flavan-3-ols. Clinical evidence on flavanones was centred around naringenin and hesperidin or their corresponding food sources. Naringenin has been found to alleviate NAFLD, TC, TG, LDL-C and HDL-C, whereas hesperidin decreased alanine aminotransferase (ALT), aspartate aminotransferase (AST), TC, TG and HOMA-IR(Reference Yang, Chen and Zhang42). However, two independent meta-analyses reported conflicting results with one showing that hesperidin did not improve lipid profiles and BP(Reference Mohammadi, Ramezani-Jolfaie and Lorzadeh43), while the other failed to come to a definitive conclusion(Reference Pla-Pagà, Companys and Calderón-Pérez44). Primarily from soy and soy products, isoflavones have been shown to improve arterial stiffness, TC, TG, LDL-C, HDL-C, fasting glucose, glucose homeostasis and HOMA-IR(Reference Glisic, Kastrati and Gonzalez-Jaramillo45–Reference Tokede, Onabanjo and Yansane47). As for flavonols, they have been shown to have a positive impact on SBP, DBP, TC, TG, LDL-C, HDL-C and fasting glucose(Reference Menezes, Rodriguez-Mateos and Kaltsatou48). Two meta-analyses on the individual flavonol, quercetin, independently revealed a significant reduction in both SBP and DBP(Reference Huang, Liao and Dong49,Reference Serban, Sahebkar and Zanchetti50) . Supplementation of lignans and lignan-rich foods has the potential to ameliorate SBP, DBP, TC, TG and LDL-C(Reference Khalesi, Irwin and Schubert51–Reference Sun, Ren and Zhu55). The majority of clinical work conducted on stilbenes revolves around resveratrol. Several meta-analyses found a link between resveratrol consumption and improvement of FMD, serum vascular adhesion molecules (ICAM-1), SBP, TC, fasting glucose, HbA1c, AST and ALT(Reference Abdelhaleem, Brakat and Adayel56–Reference Soltani, Sharifi-Zahabi and Sangsefidi60). However, it is important to note that most of these positive outcomes were only significant in unrealistically high doses (>300 mg/d). At the current standing, there is an increasing number of RCTs and meta-analyses suggesting that these (poly)phenol subclasses may have a role in supporting cardiometabolic health.
Much like observational studies, clinical interventions are certainly not exempt from notable shortcomings. Many intervention studies today still fail to fully characterise their intervention products, making it difficult to distinguish the responsible bioactives, further bringing their efficacy into question. The (poly)phenol amounts administered varied wildly across the current RCT landscape. On the higher end, dosage surpassed dietary achievable amounts, a clear example of this is the resveratrol studies administering up to >1000 mg/d on the treatment arms(Reference Abdelhaleem, Brakat and Adayel56,Reference Soltani, Sharifi-Zahabi and Sangsefidi60,Reference Fogacci, Tocci and Presta61) . Such levels are unlikely to be achievable in a normal diet, making it problematic when extrapolating results. The current lack of dose-response studies on many individual (poly)phenols and their subclasses also adds to the difficulty in determining the minimum effective dose, which is important for dietary recommendations. A number of studies have shown a temporal association between circulating plasma (poly)phenol metabolites and improvements in blood vessel function(Reference Iglesias-Aguirre, Cortés-Martín and Ávila-Gálvez62). Though promising, a causal relationship cannot be confirmed in this way. Our in vivo animal model demonstrated that physiologically relevant circulating phenolic metabolites mixtures, when administered intravenously, led to an increase in FMD(Reference Rodriguez-Mateos, Istas and Boschek38). The metabolite mixes used here were the same as the metabolites that correlated with the acute and sustained increases of FMD observed in humans. The scarcity of interventions with purified compounds limits the ability to pinpoint direct cause-and-effect, but on the other end of the conundrum, food matrix effects and the synergistic interaction between (poly)phenols and other food components should not be downplayed, as previously discussed. The current state is arguably lacking both aspects and therefore continuous efforts are highly encouraged in both directions. This emphasises the need to pair both the health benefits and metabolism together rather than separately when it comes to human intervention trials. On the technical side, the analytical evaluation of (poly)phenol, especially in biofluids, currently faces issues regarding reference standards. Many reference standards for (poly)phenol metabolites do not yet commercially exist. The only way to measure accurately these metabolites would be to synthesize in-house analytical standards which requires significant trial-and-error, time and resources, or to resort to performing enzymatic hydrolysis prior to analysis for indirect estimation. Neither solution is optimal, and this remains a major obstacle to understanding the bioavailability and kinetics of ingested (poly)phenols, simultaneously hindering efforts in validating analytical methods.
Nevertheless, the clinical evidence on the cardiovascular health benefits of (poly)phenols is stronger for flavan-3-ols than the other subclasses. This has led to the publication of the first dietary guidelines for (poly)phenols by the US Academy of Nutrition and Dietetics, with a recommendation of daily consumption of 400–600 mg of flavan-3-ols for optimal cardiometabolic health(Reference Crowe-White, Evans and Kuhnle63). This amount is easily obtained within a normal diet, achievable for example through two cups of tea, a small bowl of berries and an apple per day. It is likely that more initiatives will develop in coming years to assess the feasibility of developing dietary recommendations for plant food bioactives, such as ongoing work by the Phytochemicals and Health Special Interest Group of the UK Nutrition Society.
In terms of which (poly)phenol-rich food sources may be more beneficial, tea, berries and cocoa products are among the foods that have been more widely investigated. However, other foods widely consumed are also rich in (poly)phenols including nuts, soy products, apples and pears, coffee, and citrus fruits (Reference Ziauddeen, Rosi and Del Rio64). When comparing the efficacy of (poly)phenol supplements including (poly)phenol-rich extracts v. their corresponding whole food sources, a recent meta-analysis suggests that both whole food and purified extracts can be efficacious in reducing cardiometabolic risk(Reference Kiyimba, Yiga and Bamuwamye65), although more work is needed in this area.
Mechanisms of action: from antioxidants to modulators of the gut microbiota
As a result of findings emanating from epidemiological studies in the 90s highlighting the associations between flavonoid-rich diets and decreased mortality(Reference Hertog, Feskens and Hollman66), an over-simplification of the oxidative stress theory was used as the potential mechanism of action regarding the biological effects of (poly)phenols(Reference Ghezzi, Jaquet and Marcucci67). Indeed, as (poly)phenols demonstrated antioxidant effects in vitro, it was believed that beneficial physiological effects in humans were related to this antioxidant hypothesis(Reference Dragsted68,Reference Rice-Evans69) . This concept was followed by the development of several assays to assess the antioxidant capacities of beverages and foods in vitro, such as the 2,2-diphenyl-1-picrylhydrazyl assay, the Oxygen Radical Absorbance Capacity assay, or the Trolox Equivalent Antioxidant Capacity assay(Reference Litescu, Eremia and Radu70). However, with the advancement of the knowledge related to oxidative stress, as well as the growing scientific literature linked to the complex ADME and bioavailability of phenolic compounds in vivo, the idea of (poly)phenol effects on health to be associated merely with their antioxidant potential has been abandoned over the years(Reference Ruskovska, Maksimova and Milenkovic71). Hollman and colleagues nicely summarised that, based on the evidence available, the hypothesis that the health benefits of (poly)phenols are solely attributed to their antioxidant properties must be rejected due to the poor absorption of phenolic compounds and their extensive metabolism into derivatives with low antioxidant potential(Reference Hollman72). In addition, due to the low concentration of (poly)phenols in plasma compared to well-known antioxidants such as vitamin C or uric acid, the possibility that (poly)phenols act as direct antioxidants in vivo to improve cardiovascular health is unlikely. Although the literature related to the antioxidant activity of (poly)phenols in vitro is abundant, RCTs with relevant biomarkers of oxidative stress have failed to demonstrate such an action in vivo (Reference Halliwell, Rafter and Jenner73,Reference Hollman, Cassidy and Comte74) . One reason could be that phase II metabolism occurring in vivo leads to a decrease in the antioxidant capacity of metabolites(Reference Pollard, Kuhnle and Vauzour75). Another reason behind this divergence in findings could be, along with the common use of unmetabolized (poly)phenols, the high and non-physiologically relevant doses employed in these in vitro studies, which can be up to 100 thousand times higher than biological plasma concentrations(Reference Manach, Scalbert and Morand17). Moreover, animal studies do not account for the differences in metabolism between the investigated species and humans, which are substantial. For example, Borges et al. demonstrated that the metabolism of (–)-epicatechin in rats was very different to humans as they reported a profile of structural-related (–)-epicatechin metabolites widely different between the two species(Reference Borges, Ottaviani and van der Hooft76).
While the mechanisms of action of (poly)phenols have not still been fully elucidated, a more plausible explanation for their action is that the biological activity of (poly)phenols is exerted through the modulation of several cell signalling pathways, leading to enhanced antioxidant protection against the development of CVD(Reference Yahfoufi, Alsadi and Jambi77). In three decades, (poly)phenols went from simple antioxidant molecules to complex metabolites and signalling bioactive compounds(Reference Croft78). For example, the modulatory effects of (poly)phenols on vasodilation and the vascular tone via nitric oxide (NO) signalling pathway have been well established. They can interact with signalling pathways involving kinases such as phosphatidylinositol 3-kinase (PI3K)/Akt and eNOS, leading to an increase in NO production(Reference Vauzour, Rodriguez-Mateos and Corona79). Upon release by the endothelium, NO stimulates the soluble guanylate cyclase (sGC) in vascular muscle cells, leading to a rise in the intracellular concentration of cyclic guanosine 3′,5′-monophosphate (cGMP) and relaxation. Subsequently, cGMP can activate the protein kinase G, which can then induce the phosphorylation of other proteins to finally induce vasodilation(Reference Bian and Murad80), hence promoting blood flow(Reference Ghimire, Altmann and Straub81). Because of this, FMD has been established as a strong surrogate marker of CVD risk due to its high dependency on eNOS activity(Reference Sies82). Apart from acting as a signalling molecule, NO may also react with a superoxide anion to form peroxynitrite (ONOO-), a strong oxidant that can lead to protein and DNA damage. As NO reacts with superoxide anion O2 -faster than the rate of dismutation and inactivation of O2 - by superoxide dismutase (SOD), this concentration of SOD can be a significant determinant of the NO bioactivity(Reference Faraci and Didion83).
The activation of the nuclear E2-related factor 2 (Nrf2) receptor has arisen as a potential target for (poly)phenols in the recent literature(Reference Satta, Mahmoud and Wilkinson84). The endothelial Nrf2 can be triggered via increased reactive oxygen species production and PI3K-Akt signalling induced by shear stress(Reference Chen, Varner and Rao85). When laminar shear stress is perturbed at curved sections of arteries, the bioavailability of NO decreases, leading to an increase of the O2 - levels(Reference Hosoya, Maruyama and Kang86) and a decrease of Nrf2-activated genes, which results in a predisposition of the endothelium to atherogenesis(Reference Cheng, Siow and Mann87). The Nrf2 pathway is thus considered a key modulator of the oxidant/antioxidant balance and it has been shown that (poly)phenolic compounds could induce a response in targeted cells, leading to the activation of several defence enzymes such as glutathione-S-transferase, haem oxygenase 1, quinone oxidoreductase 1 and glutamyl-cysteine ligase(Reference Satta, Mahmoud and Wilkinson84).
In the last decades, our knowledge of the molecular mechanisms behind the biological action of (poly)phenols has substantially grown. While the Akt/eNOS, NOX and Nrf2 pathways have been widely studied in the literature, it has been shown that phenolic compounds can modulate various cellular functions indirectly related to the redox balance regulation, such as inflammation or endothelial dysfunction(Reference Ruskovska, Maksimova and Milenkovic71). On top of their action at a transcriptional level, literature recently highlighted the potential of (poly)phenols to exert some post-transcriptional regulation of genes through the modulation of non-coding RNA’s expression, called microRNAs (miRNAs), which can behave as gene regulators(Reference Milenkovic, Deval and Gouranton88,Reference Milenkovic, Jude and Morand89) . Indeed, (poly)phenols have been shown to modulate more than 100 microRNAs(Reference Krga, Milenkovic and Morand90). Through both targeted and untargeted nutrigenomic approaches followed by bioinformatic analyses of mRNA and miRNA-target pathways, Ruskovska and team revealed that (poly)phenols modulate genes involved in processes like cell adhesion and mobility, cell signalling, inflammation, lipid metabolism and endothelial function(Reference Ruskovska, Budić-Leto and Corral-Jara91,Reference Ruskovska, Budić-Leto and Corral-Jara92) . Such behaviours were also observed in a recent nutrigenomic study involving similar bioinformatic analyses coupled with in vivo microarray analysis and in silico models(Reference Milenkovic, Rodriguez-Mateos and Lucosz93). Their microarray analysis of RNA obtained from human participants who consumed bi-daily 450 mg of cocoa flavanols revealed that the distinct patterns of differentially expressed genes were correlated with changes in endothelial function, arterial stiffness and blood pressure. Their in silico docking simulation indicates increased binding affinity of (poly)phenol metabolites to cell signalling proteins and transcription factors. Mechanistic investigations such as these are insightful, but the studies are few and have used mainly cellular models and, more rarely, animal models and humans, which indicates the need for more studies to validate the impact of phenolic compounds on gene targets.
(Poly)phenols as modulators of the gut microbiota
The gut microbiota plays an important role in the bioavailability of (poly)phenols as only a small part of the ingested (poly)phenols will be absorbed in the small intestine, while the majority will reach the large intestine and interact with colonic bacteria(Reference Marchesi, Adams and Fava94). There is a bi-directional relationship between (poly)phenols and the gut microbiota: while (poly)phenols can modulate diversity, composition and possibly functionality of the gut microbiota, intestinal bacteria in turn catabolise (poly)phenols to produce numerous smaller compounds that are usually more active and which present a better absorption than the original compounds(Reference Espín, González-Sarrías and Tomás-Barberán95).
By definition, a prebiotic is a ‘non-digestible compound that modulates the composition and/or activity of the gut microbiota when metabolised by microorganisms in the gut, thus conferring a beneficial physiological effect on the host’(Reference Bindels, Delzenne and Cani96). (Poly)phenols have the potential to modulate the gut microbiota and exert a ‘prebiotic-like’ action(Reference Marchesi, Adams and Fava94). For example, tea (poly)phenols, including catechin and its derivates, were shown to inhibit the development of detrimental bacteria such as Staphylococcus aureus, Salmonella typhimurium, Helicobacter pylori or Escherichia coli (Reference Duda-Chodak, Tarko and Satora97). In human clinical trials, some other compounds, such as anthocyanins or hydroxybenzoic acids, increased the abundance of some beneficial bacteria such as Lactobacillus spp., Bifidobacterium spp. or Faecalibacterium prausnitzii, which are responsible for the production of the short-chain fatty acid butyrate(Reference González-Sarrías, Romo-Vaquero and García-Villalba98,Reference Vendrame, Guglielmetti and Riso99) . Changes in gut microbiome composition have also been correlated to beneficial changes in cardiovascular markers in human subjects. For instance, 4-week consumption of cocoa flavan-3-ols significantly increased the abundance of Lactobacillus spp. and Bifidobacterium spp., while significantly decreasing the counts of Clostridium histolyticum (Reference Tzounis, Rodriguez-Mateos and Vulevic100). The authors also reported that these changes in the microbiome were correlated with reductions in plasma triglycerides and c-reactive protein (CRP). These results are in line with another study evaluating the effect of red wine (poly)phenol consumption for 4 weeks on microbiome and clinical biomarkers. They observed a significant improvement in cholesterol and CRP, which was correlated with the changes in Bifidobacterium spp. count(Reference Queipo-Ortuño, Boto-Ordóñez and Murri101). Moreover, previous RCTs led by our team and studying the impact of 12-week consumption of aronia (poly)phenols in healthy young men as well as prehypertensive middle-aged men and women showed a significant increase in butyrate-producer bacteria such as Anaerostipes, Lawsonibacter asaccharolyticus and Intestinimonas butyriciproducens spp.(Reference Istas, Wood and Le Sayec102,Reference Le Sayec, Xu and Laiola103) . When correlation analyses were performed between vascular function and select gut microbial genera, a correlation between FMD and Dialister spp. (Spearman ρ: 0·42), Phascolarctobacterium spp. (Spearman ρ: −0·45) and Roseburia spp. (Spearman ρ: −0·45) were observed(Reference Istas, Wood and Le Sayec102). Furthermore, in a recent systematic review investigating the modulation of the gut microbiota by (poly)phenols including 22 animal studies, abundances of Akkermansia, Roseburia, Bifidobacterium and Faecalibacterium were significantly increased following the intake of tea flavan-3-ols, soy isoflavones, naringenin, lignans, stilbenes and phenolic acids by mice and rats(Reference Alves-Santos, Sugizaki and Lima104–Reference Koutsos, Lima and Conterno109).
As previously stated, the gut microbiota can transform the intact (poly)phenols reaching the colon into smaller compounds with enhanced bioavailability. The main catabolic reactions occurring in the large intestine are reductions, cleavage and hydrolysis(Reference Espín, González-Sarrías and Tomás-Barberán95). Well-known gut microbial metabolites are urolithins (derived from ellagitannins), enterolactone and enterodiol (produced from lignans), equol and O-desmethylangolensin (ODMA) (coming from isoflavones catabolism) or γ-valerolactones (derived from flavan-3-ols)(Reference Tomás-Barberán, Selma and Espín110). In this context, and due to the high inter-individual variability in gut microbial metabolism that has been demonstrated in recent years, the notion of (poly)phenol gut metabotype has been developed. This refers to a ‘group of individuals with similar (poly)phenol gut microbial metabolising capacity’ and more recently, to ‘metabolic phenotype defined by the existence of specific metabolites derived from the gut microbiota, characteristic of the precursor polyphenol metabolism and by the associated microbial ecology in terms of composition and activity’(Reference Espín, González-Sarrías and Tomás-Barberán95,Reference Bolca, Van de Wiele and Possemiers111) . Examples include the discovery of three distinct urolithin metabotypes of ellagitannins in the general population, that have been related to the presence of specific bacteria such as Gordonibacter urolithinfaciens and Ellagibacter isourolithinfaciens (Reference Selma, Beltrán and García-Villalba112–Reference Tomás-Barberán, García-Villalba and González-Sarrías114). Metabotypes have also been reported for isoflavones with the presence of equol and ODMA producer and non-producer metabotypes among the population(Reference Frankenfeld115). Indeed, some metabotypes have been found more frequently in patients with specific diseases leading to microbiome dysbiosis such as metabolic syndrome or obesity(Reference Tomás-Barberán, Selma and Espín110), although this has not been fully confirmed in larger cohorts. The relationship between metabotypes and health is still a matter of intense debate. To date, whether producers are responders and non-producers are non-responders is unclear. More RCTs where subjects are stratified into individual metabotypes are needed to elucidate whether gut microbial metabolites are the bioactive compounds responsible for the effects.
To sum up, gut microbiota plays a very important part in the bioavailability of (poly)phenols and ultimately, in their biological activity. However, the evidence to support the beneficial effects of (poly)phenol gut microbial metabolites and/or their associated gut microbiota is still unclear, let alone their mechanisms. There is a need for larger trials investigating the impact of (poly)phenol supplementation on the composition and functionality of the gut microbiota but also studies looking into the link between gut microbial-derived compounds, associated metabotypes and cardiometabolic health. The relationship between inter-individual variability in response to (poly)phenol intake and gut microbial metabolism needs to be further investigated.
Conclusion
For the past decades, researchers have concluded that more evidence is needed to confirm the effects of (poly)phenols on human health. It is time to acknowledge that we do have significant evidence on the effects of flavan-3-ols on cardiometabolic risk reduction, coming from high-quality RCTs and subsequent meta-analysis. Substantial evidence also exists on the effects of other (poly)phenols such as anthocyanins, isoflavones, flavonols and lignans on cardiometabolic outcomes. In terms of epidemiological studies, we need to improve the methods we use to assess (poly)phenol intake and develop better methodologies to estimate intake and relationships with health outcomes. While we move on from the antioxidant era, the mechanisms of action of (poly)phenols are likely to include the modulation of multiple cell signalling pathways, such as the NO pathway, activating the Nrf2 receptor and regulating hundreds of microRNAs. A gut microbiota-mediated mechanism is also likely to exist, however how exactly the gut microbiota and (poly)phenols interact and to which extent this relationship influences their health benefits is one of the key questions that need to be answered to truly elucidate and identify which individuals will benefit the most from (poly)phenol consumption.
Acknowledgements
In accepting the Nutrition Society Silver Medal Award 2023, I would like to express my thanks to a number of people, including all past and present members of my research group, my collaborators around the world, my wonderful colleagues at King’s College London and past ones at the University of Reading, and my family, for being always there for me.
Conflict of interest
The authors report no conflicts of interest.
Authorship
A.C., M.L.S. and A.R.M. wrote the first draft. All authors contributed and approved the final version of the manuscript.