Book contents
- Movement Disorders and Inherited Metabolic Disorders
- Movement Disorders and Inherited Metabolic Disorders
- Copyright page
- Dedication
- Contents
- Contributors
- Preface
- Acknowledgments
- Section I General Principles and a Phenomenology-Based Approach to Movement Disorders and Inherited Metabolic Disorders
- Section II A Metabolism-Based Approach to Movement Disorders and Inherited Metabolic Disorders
- Section III Conclusions and Future Directions
- Appendix: Video Captions
- Index
- References
Section II - A Metabolism-Based Approach to Movement Disorders and Inherited Metabolic Disorders
Published online by Cambridge University Press: 24 September 2020
- Movement Disorders and Inherited Metabolic Disorders
- Movement Disorders and Inherited Metabolic Disorders
- Copyright page
- Dedication
- Contents
- Contributors
- Preface
- Acknowledgments
- Section I General Principles and a Phenomenology-Based Approach to Movement Disorders and Inherited Metabolic Disorders
- Section II A Metabolism-Based Approach to Movement Disorders and Inherited Metabolic Disorders
- Section III Conclusions and Future Directions
- Appendix: Video Captions
- Index
- References
Summary
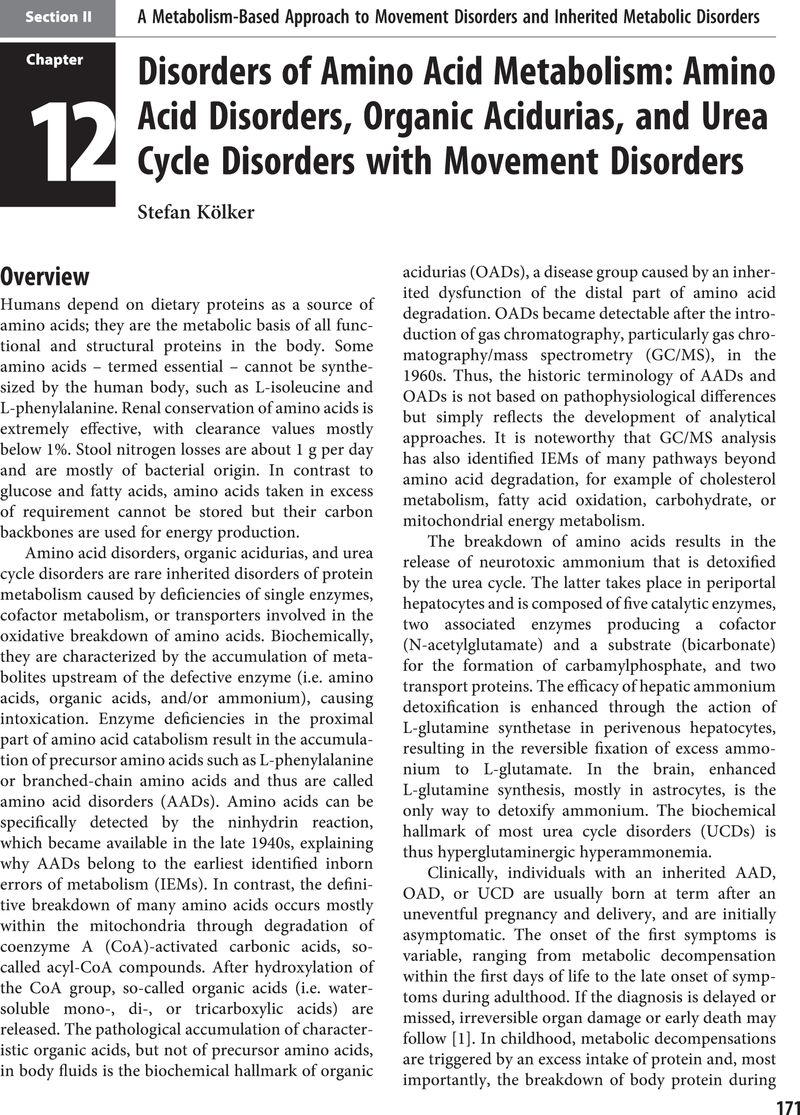
- Type
- Chapter
- Information
- Movement Disorders and Inherited Metabolic DisordersRecognition, Understanding, Improving Outcomes, pp. 171 - 364Publisher: Cambridge University PressPrint publication year: 2020