Book contents
- Adaptive Food Webs
- Adaptive Food Webs
- Copyright page
- Contents
- Contributors
- Preface
- Introduction
- Part I Food Webs: Complexity and Stability
- Part II Food Webs: From Traits to Ecosystem Functioning
- 8 Integrating Food-Web and Trait-Based Ecology to Investigate Biomass-Trait Feedbacks
- 9 Including the Life Cycle in Food Webs
- 10 Importance of Trait-Related Flexibility for Food-Web Dynamics and the Maintenance of Biodiversity
- 11 Ecological Succession Investigated Through Food-Web Flow Networks
- 12 Statistical Approaches for Inferring and Predicting Food-Web Architecture
- 13 Global Metawebs of Spider Predation Highlight Consequences of Land-Use Change for Terrestrial Predator–Prey Networks
- 14 Ecological Networks in Managed Ecosystems: Connecting Structure to Services
- 15 Trait-Based and Process-Oriented Modeling in Ecological Network Dynamics
- 16 Empirical Methods of Identifying and Quantifying Trophic Interactions for Constructing Soil Food-Web Models
- Part III Food Webs and Environmental Sustainability
- Index
- References
16 - Empirical Methods of Identifying and Quantifying Trophic Interactions for Constructing Soil Food-Web Models
from Part II - Food Webs: From Traits to Ecosystem Functioning
Published online by Cambridge University Press: 05 December 2017
- Adaptive Food Webs
- Adaptive Food Webs
- Copyright page
- Contents
- Contributors
- Preface
- Introduction
- Part I Food Webs: Complexity and Stability
- Part II Food Webs: From Traits to Ecosystem Functioning
- 8 Integrating Food-Web and Trait-Based Ecology to Investigate Biomass-Trait Feedbacks
- 9 Including the Life Cycle in Food Webs
- 10 Importance of Trait-Related Flexibility for Food-Web Dynamics and the Maintenance of Biodiversity
- 11 Ecological Succession Investigated Through Food-Web Flow Networks
- 12 Statistical Approaches for Inferring and Predicting Food-Web Architecture
- 13 Global Metawebs of Spider Predation Highlight Consequences of Land-Use Change for Terrestrial Predator–Prey Networks
- 14 Ecological Networks in Managed Ecosystems: Connecting Structure to Services
- 15 Trait-Based and Process-Oriented Modeling in Ecological Network Dynamics
- 16 Empirical Methods of Identifying and Quantifying Trophic Interactions for Constructing Soil Food-Web Models
- Part III Food Webs and Environmental Sustainability
- Index
- References
Summary
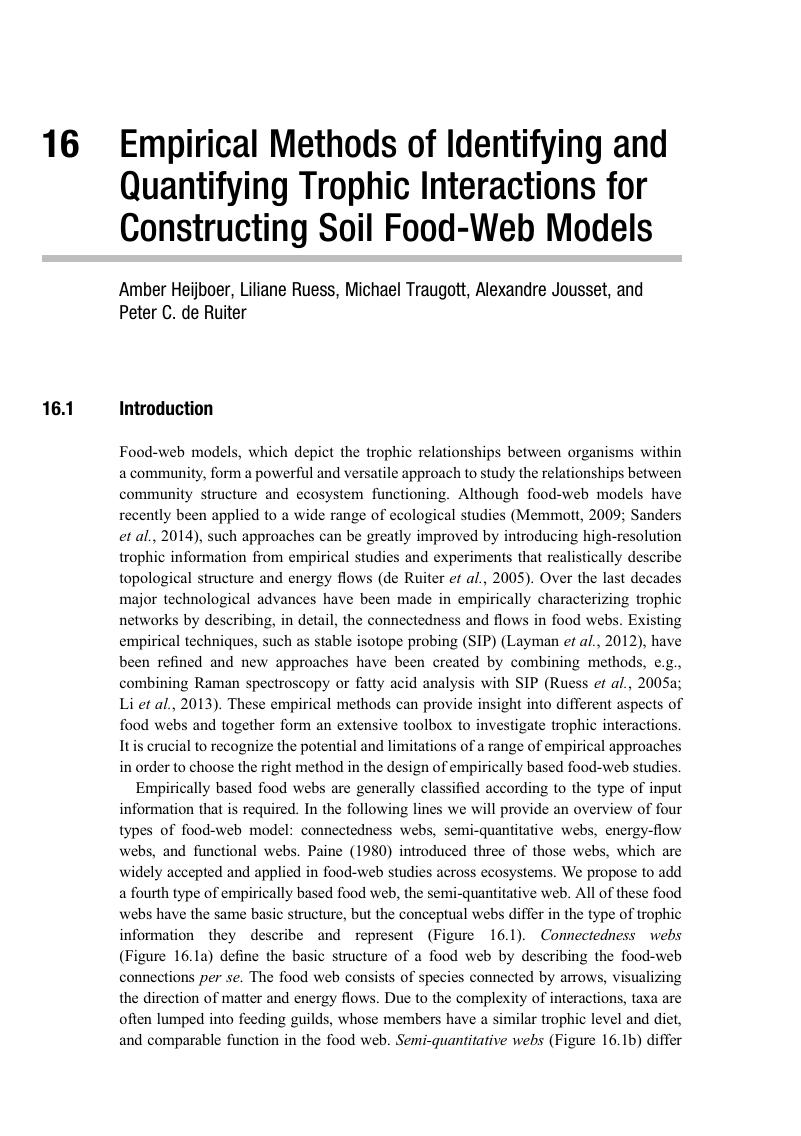
- Type
- Chapter
- Information
- Adaptive Food WebsStability and Transitions of Real and Model Ecosystems, pp. 257 - 286Publisher: Cambridge University PressPrint publication year: 2017
References
- 4
- Cited by