Book contents
- Textbook of Neural Repair and Rehabilitation
- Textbook of Neural Repair and Rehabilitation
- Copyright page
- Contents
- Contributor affiliations
- Preface
- Introduction toNeural Repair and Rehabilitation
- Section 1 Neural plasticity: cellular and molecular mechanisms of neural plasticity
- Section 2 Functional plasticity in the central nervous system
- Section 3 Plasticity after injury to the central nervous system
- Chapter 12 The role of extracellular matrix in plasticity in the spinal cord
- Chapter 13 Spinal plasticity underlying the recovery of locomotion after injury
- Chapter 14 Cellular mechanisms of plasticity after brain lesions
- Chapter 15 Pathophysiology and plasticity in cerebral palsy
- Chapter 16 Noninvasive brain stimulation in cognitive rehabilitation: guiding plasticity after injury to the central nervous system
- Chapter 17 From bench to bedside: influence of theories of plasticity on human neurorehabilitation
- Section 4 Neural repair: basic cellular and molecular processes
- Section 5 Determinants of regeneration in the injured nervous system
- Section 6 Promotion of regeneration in the injured nervous system
- Section 7 Translational research: application to human neural injury
- Index
Chapter 12 - The role of extracellular matrix in plasticity in the spinal cord
from Section 3 - Plasticity after injury to the central nervous system
Published online by Cambridge University Press: 05 May 2014
- Textbook of Neural Repair and Rehabilitation
- Textbook of Neural Repair and Rehabilitation
- Copyright page
- Contents
- Contributor affiliations
- Preface
- Introduction toNeural Repair and Rehabilitation
- Section 1 Neural plasticity: cellular and molecular mechanisms of neural plasticity
- Section 2 Functional plasticity in the central nervous system
- Section 3 Plasticity after injury to the central nervous system
- Chapter 12 The role of extracellular matrix in plasticity in the spinal cord
- Chapter 13 Spinal plasticity underlying the recovery of locomotion after injury
- Chapter 14 Cellular mechanisms of plasticity after brain lesions
- Chapter 15 Pathophysiology and plasticity in cerebral palsy
- Chapter 16 Noninvasive brain stimulation in cognitive rehabilitation: guiding plasticity after injury to the central nervous system
- Chapter 17 From bench to bedside: influence of theories of plasticity on human neurorehabilitation
- Section 4 Neural repair: basic cellular and molecular processes
- Section 5 Determinants of regeneration in the injured nervous system
- Section 6 Promotion of regeneration in the injured nervous system
- Section 7 Translational research: application to human neural injury
- Index
Summary
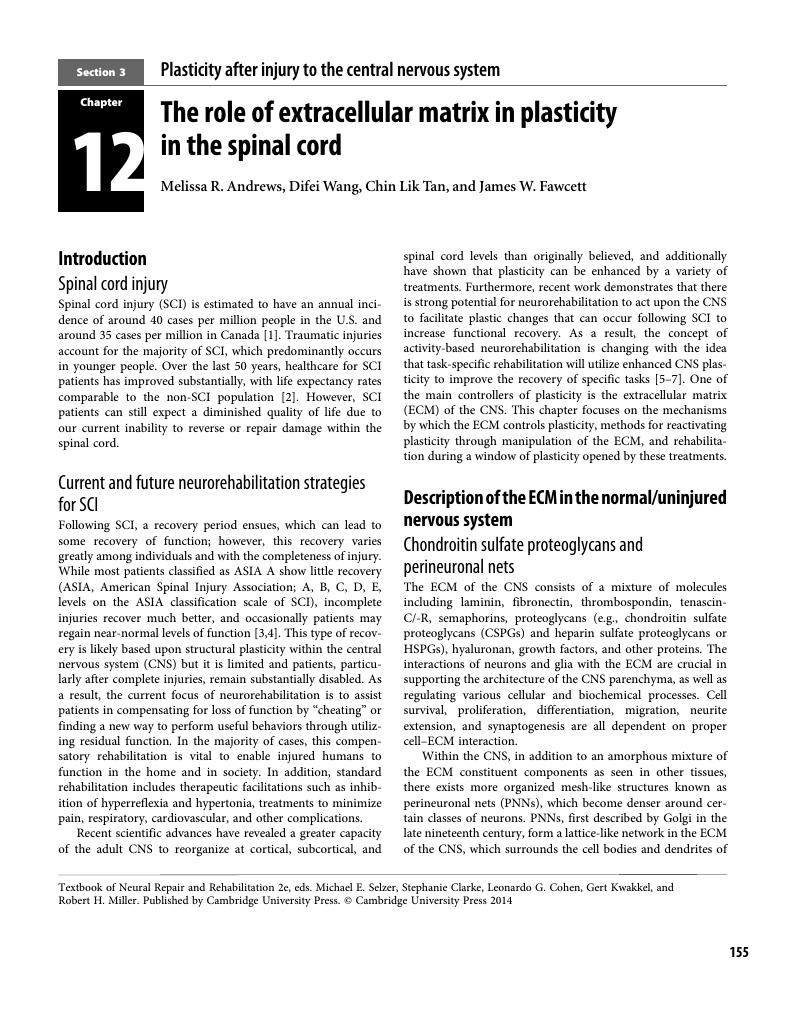
- Type
- Chapter
- Information
- Textbook of Neural Repair and Rehabilitation , pp. 155 - 165Publisher: Cambridge University PressPrint publication year: 2014