Introduction
Spectrum for high-capacity mobile backhaul applications from 92 to 114.25 GHz (commonly referred to as W-band) has been released to facilitate multi-gigabit wireless backhaul capacities for 5G and 6G [1–4]. As in the existing allocations at lower frequencies, cost and performance remain critical for the new allocation prompting the need for advanced power amplifier (PA) technology in a cost sensitive process [Reference Edstam, Hansryd, Carpenter, Emanuelsson, Li and Zirath5, 6].
Promising results at 110 GHz, and beyond, have been obtained using coplanar waveguide design in InP, GaAs, and GaN [Reference Bessemoulin, Rodriguez, Mahon, Parker and Heimlich7–Reference Camargo, Schellenberg, Bui and Estella14]. This technology is attractive due to the simplified fabrication process. However, for a given semiconductor technology, microstrip typically provides greater power [Reference Ćwikliński, Brückner, Leone, Friesicke, Mabler, Lozar, Wagner, Quay and Ambacher13, Reference Camargo, Schellenberg, Bui and Estella14].
In this paper, we present design and measurements of a pair of 90–120 GHz microstrip medium-power MMIC amplifiers, one single-ended and one balanced, fabricated using a 150-mm commercial GaAs pHEMT process. Our aim is to determine the high-frequency limits of microstrip GaAs PAs.
The paper is organized as follows: Section “Process and Amplifier Design” describes the GaAs process, and discusses transistor modeling, amplifier simulation, and design. Measurement methodology and results are presented in “Measured Results” section. These results are discussed and compared with other results in the literature in next section before some concluding remarks are made in following section.
An earlier version of this paper was presented at the 2022 European Microwave Integrated Circuit Conference, Milan, and was published in its Proceedings [Reference Mahon, Mihaljevic, Chakraborty, Gorman, Heimlich and Li15].
Process and amplifier design
Process
The process used for this work is the latest generation 0.1 µm GaAs pHEMT platform from WIN Semiconductors, PP10-20. This production technology on 150-mm wafers is optimized to support power and low noise applications through D-band and is qualified for 4 V operation. The PP10-20 transistor uses an electron beam defined 0.1 µm T-shaped gate, has typical ƒt and ƒmax of 160 and 240 GHz, respectively, and 8 dB gain at 100 GHz for a 2 × 25 µm microstrip device. The process uses a 50 µm final substrate thickness, 565 pF/mm2 capacitors, two front side interconnected metals and offers optional DC/RF isolated through-substrate via holes for low-inductance RF transitions.
Transistor modeling
Designing at a significant fraction of ƒt requires care in the transistor device model. At these high frequencies, coupling becomes significant. The approach adopted here enables (i) non-standard, non-Process Design Kit device geometries to be used and (ii) the circuit electromagnetic (EM) simulation to consider the device metallization and surrounding matching circuitry as one.
The single-finger modeling approach is used to address this issue [Reference Mahon, Dadello, Vun, Tarazi, Young, Heimlich, Harvey and Parker16]. It allows the transistor’s metal structure to be treated as part of the overall metallization, while the transistor’s active semiconductor region is modeled separately.
To extract the intrinsic semiconductor region, simple 2 × 25 µm test transistors were measured up to 50 GHz. Our MQFET model [Reference Parker17] is fitted to the measured, extracted IV, and 50 GHz S-parameters over bias. Extracted correctly [Reference Parker18], the resulting model, when used with the single-finger approach, extrapolates to different device sizes and to the high frequencies used here. The model was checked for proper scaling with respect to transistor width and number of gate fingers with fresh S-parameter data measured to 110 GHz.
Amplifier simulation
The amplifier was designed in small sections using standard microstrip elements then gradually incorporated into a full two-and-a-half-dimension EM simulation of the circuit. Figure 1 illustrates the full EM of the circuit and a close-up of a pair of output transistors modeled using the internal (gap) differential source-gate and drain-gate port technique [Reference Mahon, Dadello, Vun, Tarazi, Young, Heimlich, Harvey and Parker16].
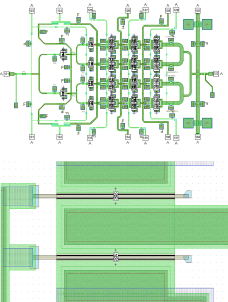
Figure 1. Upper: Core of the single-ended amplifier in Cadence Axiem EM software. The FET model is attached to the metallization using internal (gap) ports. Lower: Zoom in on part of the upper right FET in the output stage showing the internal (gap) differential source-gate and drain-gate ports.
Microstrip PA design at these frequencies is challenging as the 50-µm substrate thickness becomes a significant fraction of a wavelength at the harmonics generated by the transistor’s non-linear response. At 115 GHz, the substrate thickness is around 7% of the dielectric wavelength. At the third harmonic, it approaches a quarter wavelength and excitation of the dielectric transverse magnetic (TM) mode near 380 GHz [Reference Steer19]. In this work, only three harmonics were used in the circuit analysis instead of five as commonly used at lower frequencies.
Amplifier design
Two 90–120 GHz amplifiers have been designed. The core design is a five-stage single-ended amplifier with gate and drain biases for stages 1A and 1B connected to common gate and drain external pads, creating for the user a four-stage amplifier – see Fig. 2. The transistor peripheries are as follows, with the larger FET models extrapolated from the base device:

Figure 2. Single-ended amplifier, MMIC1. The area is 2.5 × 1.7 = 4.25 mm2.
Stage 1A: two 2 × 25 µm FETs = 100 µm,
Stage 1B: four 2 × 25 µm FETs = 200 µm,
Stage 2: four 4 × 20 µm FETs = 320 µm,
Stage 3: four 4 × 30 µm FETs = 480 µm, and
Stage 4: four 4 × 40 µm FETs = 640 µm.
The nominal bias of 250 mA/mm is obtained from a 4 V drain supply with a gate bias of −0.3 V. A conservative approach was initially taken as this was the first use of a new transistor model and the first design in a new semiconductor process.
Virtual, internal open circuits are realized in stages 1B, 2, 3, and 4 with open circuit bias lines, terminated with resonated short-circuits at center-band, supplying dc. Large de-Q’ed capacitors are incorporated into the bias supply lines that dampen low frequency gain. Matching circuits use cap-on-via to improve performance and transistor layouts with the maximum number of backvias were chosen to reduce source inductance.
An E-band PA in the same core process, with similar, small, device widths, maximum backvias, and the same dc power density of 1 W/mm was estimated to have 137°C gate temperature averaged to spot diameter of 8 µm with a backplate of 85°C [Reference Bessemoulin, Tarazi, Rodriguez, McCulloch, Parker and Mahon20]. This amplifier is expected to heat similarly.
Amplifier stability was confirmed using Rollett’s k-factor [Reference Rollett21] and Ohtomo’s loop analysis [Reference Ohtomo22]. Attention was paid to the shared bias of stage 1 and odd-mode operation for stages 2–4.
The single-ended MMIC1 layout is symmetric to facilitate construction of the second, balanced design, MMIC2, to increase the output power (Fig. 3). The Lange couplers were carefully constructed to maintain performance on the 50-µm substrate while adhering to the design rules.

Figure 3. Balanced amplifier, MMIC2. The area is 2.75 × 3.0 = 8.25 mm2.
Measured results
Vector measurements
The S-parameters were measured with an Anritsu VectorStar, using on-wafer structures for calibration. The measured gain and return losses are plotted in Fig. 4 for both the single-ended and balanced PAs. The simulated gain is also shown for the single-ended case.

Figure 4. Measured S-parameters for the single-ended (solid lines) and balanced (dashed) PA: S11 (blue), S21 (red), and S22 (green). Vd = 4 V and Vg = −0.3 V. Simulated S21 for the single-ended PA also shown (red, solid line with circles).
The gain of the single-ended and balanced amplifiers is very similar and exceeds 15 dB from about 82 to 118 GHz. The input return loss is significantly improved by the input Lange coupler as expected; however, the output return loss is only improved for some frequencies and at others it is worse, perhaps due to coupling from adjacent drain lines.
Scalar measurement methodology – WR6.5 and WR10
The scalar measurements used two different set-ups, W-band, below 110 GHz, using WR10 waveguide, and D-band, above 110 GHz, using WR6.5 waveguide. Both used Virginia Diodes Inc. (VDI) SGX series multipliers: times six for 75–110 GHz and times twelve for 110–130 GHz measurements. The former is specified as having 10 dBm minimum, 14 dBm typical output power, while the latter is specified as having 3 dBm minimum, 8 dBm typical, output power.
As more power was available at W-band, the W-band measurement input side waveguide assembly included an isolator, manual level setting attenuator and a directional coupler (with a Keysight W8486A waveguide power sensor at the couple port) between the source and the input side waveguide probe. At D-band, because the available input power was much lower and waveguide losses higher, two sets of power measurements were made: (i) over a lower input power range with a manual attenuator and directional coupler so the input power could be varied and monitored, and (ii) at a fixed, maximum possible input power.
At W-band, the output power was measured using a second Keysight waveguide power sensor. At D-band, a VDI Erikson PM5 power meter and sensor was used. This power meter is specified for operation from 75 to greater than 3 THz. The sensor uses WR-10 waveguide and tapers are available for waveguide bands above this. The total waveguide loss of the waveguide ahead of the power sensor taper must be accounted for in the measurement results. When using the W-band to D-band taper, this loss is 0.31 dB.
The measurement calibration was made in three steps. First step was to measure the power at the end of input side waveguide. At W-band, both the power at the input to the input probe and at the power at the directional coupler coupled port, were measured; at D-band, only the power at the input to the input probe was measured. The output side waveguide assembly was then added to the input side waveguide, and the power at each frequency again recorded. From this, the loss of the output side wave assembly was derived. Then, the waveguide assemblies were connected to their input and output probes, probed down on a wafer through, and the frequency steps repeated. The input probe plus through plus output probe loss was then calculated using the previous measurements. Half this loss value was then assigned to the input side, and half to the output. From these measurement steps, the power at the circuit’s input and output can be derived during the measurement. Doing the calibrations in this order meant that the waveguide connections are were not disturbed between calibration and measurement, improving the measurement integrity.
For the lower input power measurements, D-band isolators were included between the directional coupler through port and the input probe, and between the coupled port and a Keysight W8486A W-band power sensor used for input power monitoring. This ensured that the directional coupler ports saw constant D-band impedances during both calibration and measurement, despite there being a W-band-D-band mismatch at the power sensor connection. The Keysight power sensor calibration value for 110 GHz was used for all measurements, with the calibration difference between the Keysight power reading and the Erikson power meter then used to determine the input power to the circuit during measurement. For the higher input power measurements, the manual attenuator and directional coupler were removed, and the full, available input used throughout.
The D-band (WR6.5) measurement set-up is illustrated in Fig. 5. The W-band (WR10) set-up is similar. The RF probes are 100 µm waveguide Picoprobes from GGB.

Figure 5. Upper: Full WR6.5 measurement set-up with variable attenuators and sniffer. Lower: Striped down set-up without these for maximum input power.
Measured results – W-band (WR10)
The measured W-band output power is shown in Fig. 6 as a function of frequency at three gate biases, −0.5, −0.4, and −0.3 V, at the input level shown in the inset. Simulated value is also shown.

Figure 6. Output power for the single-ended PA, MMIC1, at 5 dBm wanted input power. Vd = 4 V; Vg = − 0.5 V (blue), −0.4 V (green), and −0.3 V (red). Red, solid line with circles is simulation for − 0.3 V. Actual power at the DUT when 5 dBm is the wanted input power is shown in the inset—it falls rapidly above 108 GHz with the W-band (WR10) experimental set-up.
As with the S-parameter simulation, the transistor model has not been refitted to FET data from the amplifier’s wafer nor has any other parameter been adjusted such as MIM capacitance value (i.e. SiNx thickness). The simulation is the original design prediction based on measured 50 GHz 2 × 25 µm S-parameter data. Based on Figs. 4 and 6, the discrepancy between measurement and simulation is a shift of about 5 GHz. The gain and power levels are in good agreement.
Measured scalar gain, output power, and power-added efficiency (PAE) for the single-ended amplifier at 108 GHz are shown in Fig. 7 for gate biases −0.5, −0.4, and −0.3 V. The P1dBs are, respectively, 12.9, 16.5, and 17.4 dBm. The P3dB point was not reached for the −0.5 V gate bias but is 18.7 dBm for both higher biases and the maximum output power is 20.4 dBm. The maximum PAE is not reached. The value measured here varies between 4.4% and 5.3%.

Figure 7. 108 GHz single-ended, MMIC1, measured output power (solid), gain (dashed), and PAE (dot-dash) vs input power. Bias: Vd = 4 V; Vg = − 0.5 (blue), −0.4 (green), and −0.3 V (red). Dots mark exact measurement points.
Figure 8 shows the measured power and PAE of the balanced amplifier. The output power reaches 22.5 dBm at 108 GHz and exceeds 20 dBm from 86 to 112 GHz. Figure 9 shows the data vs input power. The P1dB for the −0.3 V bias is 21.0 dBm and the P3dB is 22.3 dBm; both 3.6 dB higher than single-ended case. Again, the maximum PAE is not reached but exceeds 4.1%.

Figure 8. Output power (circles) and PAE (triangles) for the balanced PA, MMIC2, at 5 dBm wanted input power (see Fig. 2). Vd = 4 V and Vg = − 0.5 V (blue), −0.4 V (green), and − 0.3 V (red).

Figure 9. 108 GHz balanced, MMIC2, measured output power (solid), gain (dashed), and PAE (dot-dash) vs input power. Bias: Vd = 4 V; Vg = −0.5 (blue), − 0.4 (green), and −0.3 V (red). Dots mark exact measurement points.
The Lange couplers used in the balanced amplifier have performed well despite the high frequency and thin substrate. There is little loss to the measured gain and the balanced P1dB and P3dB are a little more than 3 dB higher than in the single-ended case. We suggest that the load-presented to core amplifiers by the Lange coupler in the balanced case provides a better power match than provided by the measurement probe and instrumentation in the single-ended case.
Measured results – D-band (WR6.5)
Only a modest range of input powers were achievable under attention control with the WR6.5 (D-band) system, so an additional maximum input power point is added by removing the input power coupler and sniffer. This extends the available input power from approximately −8 dBm at the DUT to 0–4 dBm. Figure 10 shows the measured 110–120 GHz gain and output power as the input power is controlled plus at an additional maximum input power point. Clearly, MMIC1 begins to compress before this final input power level is reached.

Figure 10. MMIC1 output power and gain vs input power at 110 (blue), 115 (green), and 120 GHz (red). Bias: Vd = 4 V; Vg = − 0.2 V.
The optimal bias is explored in Figs. 11 and 12, where it is determined that a lower drain bias, such as Vd = 2.5 V, and higher gate bias, such as Vg = −0.1 V, maximizes output power at these higher frequencies. This improved 120 GHz performance at the lower drain potential/higher current biases may be due to the higher gain in intermediate stages at the lower drain/higher current bias.

Figure 11. 120 GHz MMIC1 and MMIC2 output power vs gate bias. Vd = 2.5 V.

Figure 12. 120 GHz MMIC1 and MMIC2 power vs drain bias. Vg = − 0.2 V.
Figure 13 plots the output power of MMIC1 and MMIC2 at this optimal bias of Vd = 2.5 V and Vg = −0.1 V as a function of frequency for the maximum achievable input power. At 120 GHz, the output power is 12.6 dBm with a PAE of 1.2% for MMIC1 and 17.4 dBm with a PAE of 1.8% for MMIC2. The modest saturation exhibited in Fig. 10 suggests that higher powers are achievable for both MMICs, and similarly the maximum PAE value may be achieved at a different input power level than the maximum experimental input power achieved here. The 5.8 dB difference between the output powers of MMIC1 and MMIC2 at 120 GHz is presently unexplained.

Figure 13. MMIC1 (blue) and MMIC2 (red) output power vs frequency at maximum achievable input power (green) in our laboratory with this D-band (WR6.5) experimental set-up. Bias: Vd = 2. 5 V and. Vg = − 0.1 V.
The 120 GHz output power levels are less than expected from the initial single-ended simulation in Fig. 6 despite the input difference, i.e. 20.7 dBm simulated with 5 dBm input power compared with 12.6 dBm measured with 1.85 dBm input power. This may be due to inaccuracies with the scalable model. It may also be a result of only simulating to the third harmonic or the influence of the dielectric TM mode on higher harmonics.
Comparison and discussion
The work presented here is compared with selected state-of-the-art microstrip and coplanar GaAs, InP and GaN FET amplifiers at 120 GHz in Table 1.
Table 1. Selected microstrip and coplanar 120 GHz FET amplifiers

a 138 GHz (Pin = 0 dBm).
b PAE = 1.4–2.6% over 105–115 GHz.
c PAE = 1–5% elsewhere.
d 5% at 108 GHz.
e 3–8% for 90–112 GHz.
The cascode coplanar designs in [Reference Bessemoulin, Rodriguez, Mahon, Parker and Heimlich7] were fabricated on an earlier generation of the WIN PP10 process used here. The reference does not report the power at 120 GHz; however, the 7–8 dBm reported at 138 GHz is likely to be similar at 120 GHz given the flatness of the gain.
In [Reference Samoska and Leong8], the coplanar design fabricated at HRL obtains more than 9 dB of gain and 14–16 dBm of output power over 70–140 GHz from a 100-nm InP process. The authors report a left shift between simulated and measured gain of about 10 GHz.
The higher breakdown and, hence, drain bias of GaN PAs is associated with enhanced PAE, although this may be reduced in practice by higher knee voltage and knee walkout.
The F-band, 5-stage cascode CPW design from Raytheon in [Reference Fung, Samoska, Kangaslahti, Lin, Mehdi, Sadowy, Tanelli, Esteban-Fernandez, Peralta, Soria and Brown9] achieves an output power of 17.4 dBm and PAE of 4.3% at 120 GHz but these metrics are not reported for other frequencies. The measured gain roll-off either side of 120 GHz suggest that both the output power and PAE may be reduced.
The gain of the four-stage, 100-nm GaN cascode CPW design in [Reference Weber, Cwiklinski, Wagner, Lozar, Massler, Brückner and Quay10] peaks strongly at 110 GHz; however, the reported saturated output power is relatively flat, varying from 18.2 dBm at 105 and 18.5 dBm at 120 GHz to 20.3 dBm at 115 GHz. The PAE varies from 1.4% at 105 GHz to 1.7% at 120 GHz, peaking at 2.6% at 115 GHz.
The cascode CPW design in [Reference Thome, Brückner, Leone and Quay11] is fabricated on a shorter 70 nm gate length GaN process. It achieves output power above 22 dBm and PAE of 10%, or better, from 89 to 123 GHz. The measured S-parameters and power are in good agreement with simulation.
The D-band 100-nm GaN cascode CPW design reported in [Reference Ćwikliński, Brückner, Leone, Friesicke, Mabler, Lozar, Wagner, Quay and Ambacher13] has more than 25 dB of gain from 107 to 148 GHz. Excellent output power, 26.5 dBm at 15 V and 25.0 dBm at 10 V, and PAE, 11.5% and 16.5% respectively, are reported at 120 GHz. However, the output power and PAE match fall away sharply at other frequencies with the PAE dropping to near 1%.
The common source, microstrip GaN designs reported by Quinstar, and fabricated by HRL, in [Reference Camargo, Schellenberg, Bui and Estella14] exhibit excellent output power and leading PAE over a broadband of 102–118 GHz for their D1 design and 98–122 GHz for D2. The authors compare the measured and simulated S-parameters with the simulations in reasonable agreement although some frequency left shift, lower gain, and unsimulated gain notches are observed.
The PAs presented here, fabricated on a commercial 6-inch process, produce the highest power yet reported by GaAs MMICs at 120 GHz. Furthermore, they produce comparable gain, power, and PAE to designs in more expensive processes, such as InP and GaN across the 90–120 GHz range.
Conclusion
A single-ended and a balanced 90–120 GHz microstrip PAs have been designed in a new, commercial 150-mm (6-inch), 0.1-µm GaAs process for cost-sensitive W- and D-band communications applications.
At 108 GHz, measured output power is 20.4 and 22.5 dBm, respectively. At 120 GHz, measured output power is 12.6 and 17.4 dBm, respectively. Good agreement with original simulation has been shown for the lower, W-band, range, but the agreement is poorer for D-band.
These output powers and PAEs are the highest reported for GaAs and among the highest reported to date for microstrip amplifiers at these frequencies. Fabricated in an inexpensive, commercial, 6-inch GaAs pure-play foundry, they are competitive with circuits fabricated in high-cost InP and GaN processes at non-commercial, restricted foundries.
Acknowledgements
The authors thank Andrew Jones for measurement assistance and WIN Semiconductor Corp., Taiwan, for access to PP10-20.
Author contributions
S.J.M. led the circuit design, data analysis, and paper writing. M.G.M. designed and implemented the circuit measurement. J.M. and M.C.G. made significant contributions to the circuit design. A.E.P. developed the transistor model and M.C.H. provided project guidance and funding.
Funding statement
This research received no specific grant from any funding agency, commercial or not-for-profit sectors.
Competing interests
The authors report no conflict of interest.

Simon Mahon received his Bachelor of Science in Pure Mathematics and Physics in 1985, his Bachelor of Engineering in Electrical Engineering with First Class Honours in 1987, his PhD on the non-linear modelling of GaAs HEMTs in 1993, all from the University of Sydney. In 1993, he joined the CSIRO Radiophysics Laboratory to design GaAs and InP MMICs. He co-founded startup Mimix Broadband in 2001 as Director of MMIC Design, later becoming a Distinguished Fellow of Technology after the 2010 merger with Macom. Simon joined Macquarie University as an Industry Professor in 2018. He co-founded and co-directs the Macquarie Analog Devices Laboratory, and his interests include GaAs and GaN transistor modeling and MMIC design.

MacCrae (Gerry) McCulloch received his Bachelor of Engineering degree from the New South Wales University of Technology in 1976, in Sydney, Australia. He worked with CSIRO Division of Radiophysics on several cryogenically cooled radio astronomy receiving systems from 5 to 120 GHz and the local oscillator system for the Australia Telescope. In 1991–1992, he spent 15 months on sabbatical at Nobeyama Radio Observatory in Japan. In 2001, Gerry joined the Mimix Broadband (later Macom) Sydney Design Centre, where he established the laboratory to measure MMIC circuits to 140 GHz. In 2019, he established a similar facility for the Macquarie Analog Devices Laboratory at Macquarie University.

Jakov Mihaljevic received the Bachelor of Engineering and Economics degree in 2019 and his Master of Research degree in high frequency power amplifier design in 2021, both from Macquarie University. He is employed at Macquarie Analog Devices Laboratory as a MMIC designer, and his main research interests are in novel design of high-power amplifier and switching circuits in III-V semiconductor technologies.

Melissa Gorman was born in 1983 in Maracaibo, Venezuela. She received her Bachelor of Science, in the field of Electronic Engineering, from Simon Bolivar University in Caracas, Venezuela, in 2006. She completed her Master of Science, in the field of Microwave Engineering, from Technical University of Munich in Munich, Germany, in 2009. Melissa joined the Research Department at Macom Technology in Sydney, Australia, in 2011 where she worked as an MMIC Design Engineer and developed a variety of devices from power amplifier to frequency multipliers in different frequency ranges. In 2018, she joined Macquarie University in Sydney to further her experience in MMIC design. Associate Professor Gorman is the co-director of the Macquarie Analog Devices Laboratory, and she thoroughly enjoys working with high frequency design projects and participating in the evaluation process of these designs to final products.

Emeritus Professor Anthony E. Parker received the B.Sc., B.E., and Ph.D. degrees from The University of Sydney, Australia, in 1983, 1985, and 1992, respectively. He joined Macquarie University, Sydney, in 1990 and held a professorial position within the School of Engineering, which he led during its foundation. He co-founded the Macquarie Analog Devices Laboratory (MAD Lab) in 2018 and continues to contribute as a consultant. His research focus is microwave device and circuit. He has interests in the characterization of microwave devices, including pulse testing and CAD implementations. His recent work investigates scalable models of GaAs and GaN devices. He has consulted with several companies including Macom and Agilent Technologies. He has developed accurate simulation techniques used in FET and HEMT models. This includes a transistor model development for very accurate nonlinear dynamic models of GaN HEMTs. He has authored or coauthored over 210 publications.