Introduction
During life, bone is a living tissue that records various aspects of the life history and biology of an animal. It is therefore fortuitous that despite millions of years of fossilization, the integrity of the microscopic structure of bones of fossil vertebrates remains intact (Chinsamy-Turan, Reference Chinsamy-Turan2005), thereby allowing paleobiological deductions. Although the bone histology of placental mammals has been well studied (e.g., Enlow and Brown, Reference Enlow and Brown1958; Klevezal and Kleinenberg, Reference Klevezal and Kleinenberg1969; Kolb et al., Reference Kolb, Scheyer, Veitschegger, Forasiepi, Amson, Van der Geer, Van den Hoek Ostende, Hayashi and Sánchez-Villagra2015; Montoya-Sanhueza et al., Reference Montoya-Sanhueza, Bennett, Oosthuizen, Dengler-Crish and Chinsamy2021a, Reference Montoya-Sanhueza, Bennett, Oosthuizen, Dengler-Crish and Chinsamy2021b), the osteohistology of marsupials is sorely lacking (Chinsamy and Warburton, Reference Chinsamy and Warburton2021), despite work on them having begun in the nineteenth century (Quekett, Reference Quekett1849) and early twentieth century (Foote, Reference Foote1916; Amprino, Reference Amprino1947), and continuing sporadically thereafter (e.g., Enlow and Brown, Reference Enlow and Brown1958; Singh et al., Reference Singh, Tonna and Gandel1974; Werning, Reference Werning2013; Walker et al., Reference Walker, Louys, Herries, Price and Miszkiewicz2020). Growth marks in marsupials were first reported in a large extinct diprotodontid (unknown species) (Leahy, Reference Leahy1991), and thereafter in a kangaroo (Chinsamy-Turan, Reference Chinsamy-Turan2005; Hurum and Chinsamy-Turan, Reference Hurum, Chinsamy-Turan and Chinsamy-Turan2012). The most comprehensive study of marsupial osteohistology (in terms of number of extinct and extant taxa) was done by Werning (Reference Werning2013) (although Nimbadon was not analyzed). More recently, Chinsamy and Warburton (Reference Chinsamy and Warburton2021) undertook the only osteohistology study documenting histological changes through ontogeny of the Western Grey Kangaroo, Macropus fuliginosus Desmarest, Reference Desmarest and Sonnini1817. Bone disease in wombats, Vombatus ursinus Shaw, Reference Shaw1800, has been examined by (Slon et al., Reference Slon, Stein, Cohen, Medlej, Peled and Hershkovitz2014). Walker et al. (Reference Walker, Louys, Herries, Price and Miszkiewicz2020) studied the forelimbs of extinct and extant wombats to assess their biomechanical and physiological adaptations to their fossorial lifestyle. In a study looking at limb bone scaling in macropods and quadrupedal artiodactyles, Doube et al. (Reference Doube, Felder, Chua, Lodhia, Kłosowski, Hutchinson and Shefelbine2018) speculated that the hopping behavior of macropods might have enhanced repair of fatigue damage by increasing bone remodeling. However, unexpectedly, they found that a femur of Macropus gianteus Shaw, Reference Shaw1790, lacked any bone remodeling. In a study of skeletal lesions caused by fluorosis in six species of marsupials, Death et al. (Reference Death, Coulson, Kierdorf, Kierdorf, Ploeg, Firestone, Dohoo and Hufschmid2017) found that the pathologies evident in their bones concurred with those of other fluoride-related skeletal changes described for other mammals.
Our research focuses on juvenile and adult Nimbadon lavarackorum Hand et al., Reference Hand, Archer, Rich and Pledge1993 (Zygomaturinae, Diprotodontidae, Marsupialia), that lived during the Middle Miocene (ca. 15 Ma; Woodhead et al., Reference Woodhead, Hand, Archer, Graham, Sniderman, Arena, Black, Godthelp, Creaser and Price2016) of Australia. Diprotodontids are a taxonomically (18 genera, 30 species) and ecologically diverse group of extinct, large-bodied (70–2000 kg) marsupials commonly found in late Oligocene to Pleistocene deposits of Australia and New Guinea. At ~70 kg, N. lavarackorum is regarded as one of the smallest diprotodontids, but one of the largest mammals to have occupied the forest canopies of Australia (Black et al., Reference Black, Camens, Archer and Hand2012a). Anatomically, its strong forelimbs, highly mobile shoulder and elbow joints, semi-opposable digit 1 on the manus and pes, and large recurved claws make it similar in some respects to modern koalas (Phascolarctos cinereus Goldfuss, Reference Goldfuss and von Schreber1817) and highly adapted for an arboreal lifestyle. The proportionately short hindlimbs (relative to forelimbs) of N. lavarackorum may also indicate some suspensory behavior (Black, 2012a). Its closest living relatives are wombats and koalas.
We hypothesize that the postcranial bone histology of Nimbadon lavarackorum will show growth marks in the form of alternating periods of fast and slow growth, and that lines of arrested growth (LAGs) will be associated with the periods of slow growth. Since we are examining both juvenile and adult bones of the species, we predict that the number of growth marks in the bones of N. lavarackorum will increase through ontogeny, and we expect to see a slowing down in both appositional periosteal growth and in endochondral growth (i.e., growth in length).
Materials and methods
Ten skeletal elements were studied: two juvenile femora and two juvenile tibiae (identified on the basis of dimensions and comparison with more complete specimens, as well as degree of epiphyseal fusion), three larger (probably adult) femora, and three larger tibiae (Fig. 1, Table 1). All of these were recovered from the Middle Miocene (14.82 ± 0.27 Ma and 14.64 ± 0.46 Ma; Woodhead et al., Reference Woodhead, Hand, Archer, Graham, Sniderman, Arena, Black, Godthelp, Creaser and Price2016) AL90 site, Riversleigh World Heritage Area (Boodjamulla) in Waanyi area of northwestern Queensland (project numbers, DE130100467, DP170101420). All specimens were prepared using dilute acetic acid to process limestone speleothems (flowstone) that had accumulated in a cave deposit (Arena et al., Reference Arena, Black, Archer, Hand, Godthelp and Creaser2014). Current understanding is that the paleohabitat surrounding the AL90 cave during the Middle Miocene was species-rich, lowland rainforest (Archer et al., Reference Archer, Hand, Godthelp and Hill1994; Travouillon et al., Reference Travouillon, Legendre, Archer and Hand2009, Reference Travouillon, Archer and Hand2012; Black et al., Reference Black, Camens, Archer and Hand2012a, Reference Black, Archer, Hand, Godthelp and Talentb; Arena et al., Reference Arena, Black, Archer, Hand, Godthelp and Creaser2014; Myers et al., Reference Myers, Black, Archer and Hand2017) that also contained isolated, ephemeral, internally drained lakes.
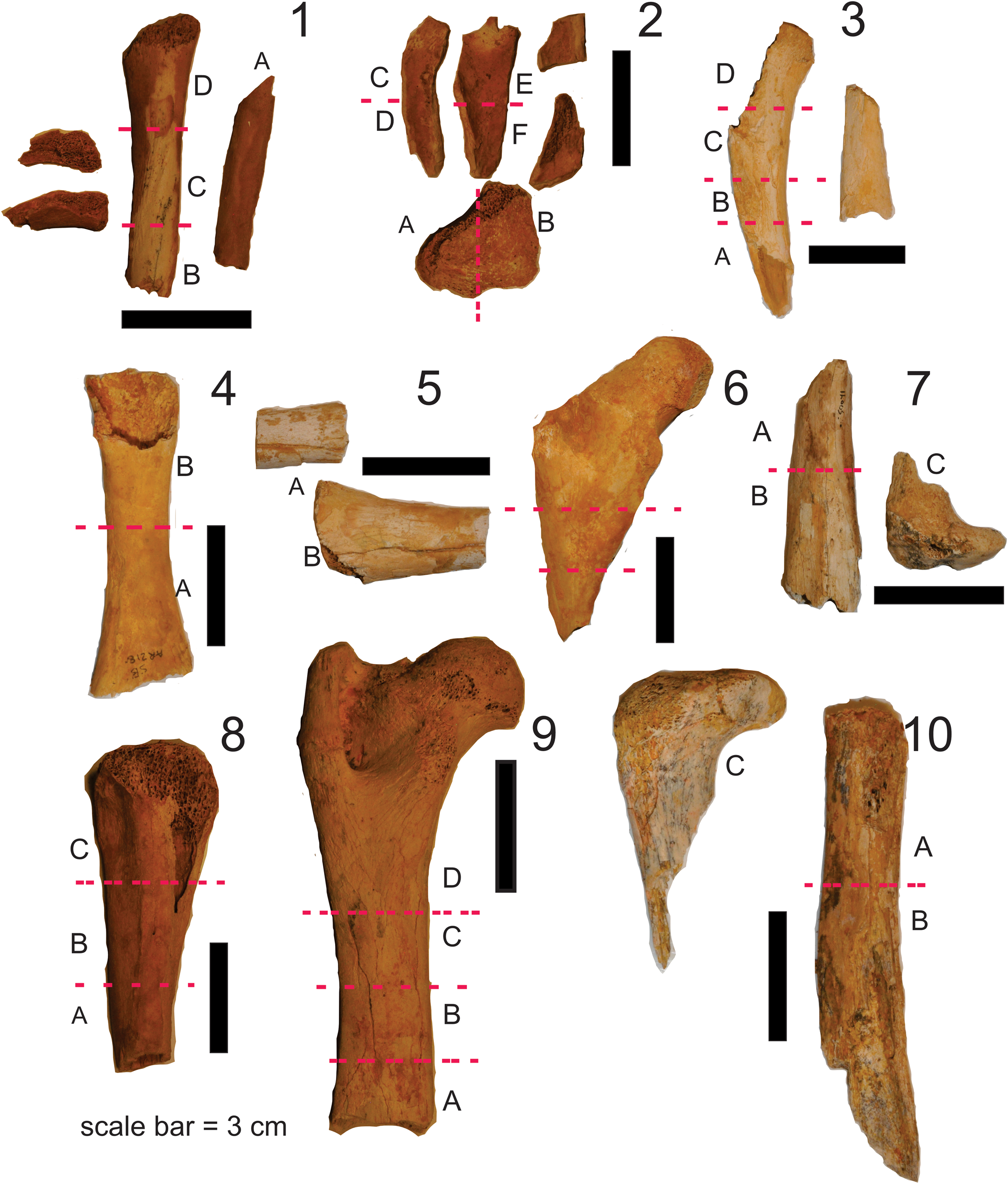
Figure 1. Nimbodon lavarackorum material used in this study. (1) AR21802a, partial juvenile tibia; (2) AR21802b, partial juvenile femur; (3) AR21803a, partial juvenile tibia; (4) AR21803b, partial femur; (5) AR21804, femur fragments; (6) AR21805, proximal femur; (7) QM F50691 partial tibia; (8) QM F41185, partial tibia; (9) QM F50842a, partial femur; (10) QM F50692, partial femur. Dashed red lines indicate where the bones were sectioned into different blocks. labeled A, B, C, etc. Abbreviations: AR, paleontological collections in the School of Biological, Earth and Environmental Sciences, University of New South Wales; QM F, paleontological collection of the Queensland Museum; a, b indicate associated material.
Table 1. Specimens studied and thin sections that were prepared from each element. Note that specimens AR21802a and AR21802b were associated and are likely from the same juvenile (juv) individual. The same applies to specimens AR21803a and AR21803b. frag = fragmentary; prox = proximal, dist = distal; TS = transverse section, LS = longitudinal section.

Prior to embedding in resin for thin sectioning, all the bones were pre-treated by leaving them in 95% alcohol for 8 hours. Thereafter, the alcohol was discharged and fresh alcohol was poured over the specimens. This was done three times and was followed by two overnight baths in acetone. After the acetone treatment, the bones were left to dry completely. The pre-treatment procedure removes any remaining organic material (such as collagen) in the bones and prevents the specimen “lifting” off the slide during thin-section preparation (Chinsamy et al., Reference Chinsamy, Angst, Canoville and Göhlich2020).
Following pre-treatment, the bones were sampled for thin sectioning (Fig. 1). Transverse sections along the diaphysis (including the midshaft) were made to obtain information about the organization of the bone along the shaft. Longitudinal thin sections were made of bones that preserved articular ends to obtain information about endochondral growth (Fig. 1). Once segments of the bones were removed, thin sections were prepared following the methodology outlined in Chinsamy and Raath (Reference Chinsamy and Raath1992). Resulting thin sections were examined under a Zeiss microscope/Nikon E200 microscope, and photomicrographs were taken using a Zeiss Axio camera/Nikon camera.
Histology terminology used generally follows the traditional bone terminology of Francillon-Vieillot et al. (Reference Francillon-Vieillot, De Buffrénil, Castanet, Géraudie, Meunier, Sire, Zylberberg, De Ricqlès and Carter1990) and Chinsamy-Turan (Reference Chinsamy-Turan2005, Reference Chinsamy-Turan2012). It should be noted that the orientation of the canals in the bone generally are used as a proxy to describe the extent and orientation of vascular canals present in the bone. However, each vascular canal can house a variable number of blood vessels, nerves, and other connective tissues (Chinsamy-Turan, Reference Chinsamy-Turan2005). It is also worth noting that the shape of the canals do not reflect the orientation of the actual blood vessels therein (Starck and Chinsamy, Reference Starck and Chinsamy2002; Chinsamy-Turan, Reference Chinsamy-Turan2005).
Growth marks (GMs) are indicated by the stratification of the compacta into alternating bands of rapidly formed bone tissue (zones), and more slowly formed bone tissue (annuli) that are often associated with lines of arrested growth (LAGs), which indicate periodic cessation of growth (Francillon-Vieillot et al., Reference Francillon-Vieillot, De Buffrénil, Castanet, Géraudie, Meunier, Sire, Zylberberg, De Ricqlès and Carter1990; Chinsamy-Turan, Reference Chinsamy-Turan2005, Reference Chinsamy-Turan2012).
Repositories and institutional abbreviations
AR, paleontological collections in the School of Biological, Earth and Environmental Sciences, University of New South Wales; QM F, paleontological collection of the Queensland Museum. All thin sections will be housed with the original collections from which the material originated.
Results
Detailed descriptions of the cross-sectional histology and, where possible, the longitudinal osteohistology of N. lavarackorum specimens follows.
AR21803a juvenile partial tibia (associated with partial femur, AR21803b)
The compacta is mostly damaged by diagenesis, but details of the histology are still evident. Several GMs interrupt the primary bone compacta (Fig. 2.1).

Figure 2. Specimen AR21803. (1) AR21803a, thin section BII; section of partial tibia; arrows indicate growth marks in the compacta; (2) AR21803b, thin section AI; section of partial femur showing a low-magnification overview of the compacta; arrows indicate growth marks in the compacta (note the resorptive endosteal margin of the bone wall); (3) AR21803b, thin section AI showing radial tract of compacted coarse cancellous bone (indicated by the white arrows). Images taken under polarized light with a one-quarter-λ compensator.
AR21803b juvenile partial femur (associated with partial tibia, AR21803a)
The bone tissue is affected by diagenesis (probably manganese infiltration), but despite this, it is evident that the compacta is well vascularized with many mainly radially organized vascular canals. The bone appears to have a more lamellar texture, and at least three growth marks are evident in the compacta (Fig. 2.2), although these are not consistently visible around the bone. The endosteal margin is highly resorptive, and several large erosion cavities are visible in the perimedullary region (Fig. 2.2). A narrow strip of endosteal lamellar bone tissue is present in some parts of the bone wall. It is notable that the bone tissue changes quite dramatically from one area of the thin section to the next. Figure 2.3 shows a radial tract of bone tissue that is composed of more compacted coarse cancellous bone (cccb) as compared to the primary lamellar bone on the one side and a more woven textured primary bone on the other side.
AR21802a juvenile tibia (associated with partial femur, AR21802b)
A partial thin section of the tibial bone wall is preserved. The compacta is well vascularized. Here, the histology is quite variable across the thin section. Several closely spaced LAGs are visible in the peripheral region of one part of the bone wall (Fig. 3.1), but if you follow the LAGs around the compacta, they then become wider apart. Also, in the latter region, the bone wall is very uneven. The outer third of the bone wall consists of primary bone tissue, but there are different types of bone tissue internal to this, of which cccb appears to be the predominant type (Fig. 3.1–3.3). Some enlarged erosion cavities and several secondary osteons are visible in the perimedullary area (Fig. 3.1). The endosteal margin is resorptive in places, but in other parts, there is a narrow band of lamellar bone tissue that lines the medullary cavity (Fig. 3.1). The longitudinal section of the bone is quite badly affected by diagenesis, but a patchy distribution of calcified cartilage is visible (Fig. 3.4).

Figure 3. Specimen AR21802. (1–4) AR21802a, partial tibia; (1) AR21802a, thin section AII; black arrows indicate growth marks in the compacta, white arrow indicates a narrow band of inner circumferential lamellar bone tissue lining the medullary cavity (note that the endosteal margin of the bone wall is resorptive); (2) AR21802a, thin section DI; arrows indicate growth marks formed by narrow annuli; the vascular canals tend to be radially organized in this part of the bone wall; (3) AR21802a, thin section CI; a different part of the bone wall showing the predominance of compacted coarse cancellous bone tissue; (4) AR21802b, partial femur, thin section D; longitudinal section showing some calcified cartilage. (5–7) AR21802b, partial femur, thin section AIV; (5) arrows indicate several LAGs that interrupt deposition of the poorly vascularized parallel-fibered bone tissue; several short Sharpey's fibers are visible towards the periosteal region of the bone wall (black arrow); a region of reconstructed secondary bone tissue (blueish color) is present below the narrow strip of primary bone; (6, 7) overview of trabecular bone tissue; (7) higher magnification of framed region in (6); arrows indicate islands of calcified cartilage in the trabeculae. Images taken under polarized light with a one-quarter-λ compensator.
AR21802b juvenile femur (associated with partial tibia, AR21802a)
This thin section also shows some diagenetic alteration, but the histology of the outer part of the cortex is visible (Fig. 3.5). There appears to be a distinct band of parallel-fibered bone tissue in the peripheral region, which is interrupted by deposition of several LAGs (Fig. 3.5). Some of the LAGs are very closely associated and could be double LAGs, but this is not certain (Fig. 3.5). The osteocyte lacunae in this region have abundant canaliculi that extend from them. Below this band of tissue, there is a wider region consisting of cccb. The longitudinal sections show cancellous bone tissue in which there are patches of calcified cartilage located in the trabeculae (Fig. 3.6, 3.7).
AR21804 femur fragment(s)
Bone is diagenetically altered. There are large amounts of a dark inclusion (probably manganese) that penetrates the bone wall. The medullary cavity seems to be infilled by a calcite-like material. The organization of the tissue that makes up the bone wall is not visible; however, it is evident that there are several vascular spaces and erosion cavities in the compacta. Thin sections of this specimen are not illustrated.
AR21805 proximal femur
Unfortunately, this section was not a proper transverse section (i.e., not taken perpendicular to the long axis of the bone), therefore the histology is not comparable to other sections. The osteocytes have been sectioned in different orientations and reveal a narrow outer compact bone wall with GMs and inner more cancellous tissue. The longitudinal section shows several rows of calcified cartilage, but this does not appear to have been fast-growing tissue because the aligned calcified cartilage rows are not numerous (Chinsamy and Warburton, Reference Chinsamy and Warburton2021). Several Sharpey's fibers are visible in the preserved articular region of the bone (Fig. 4.1).
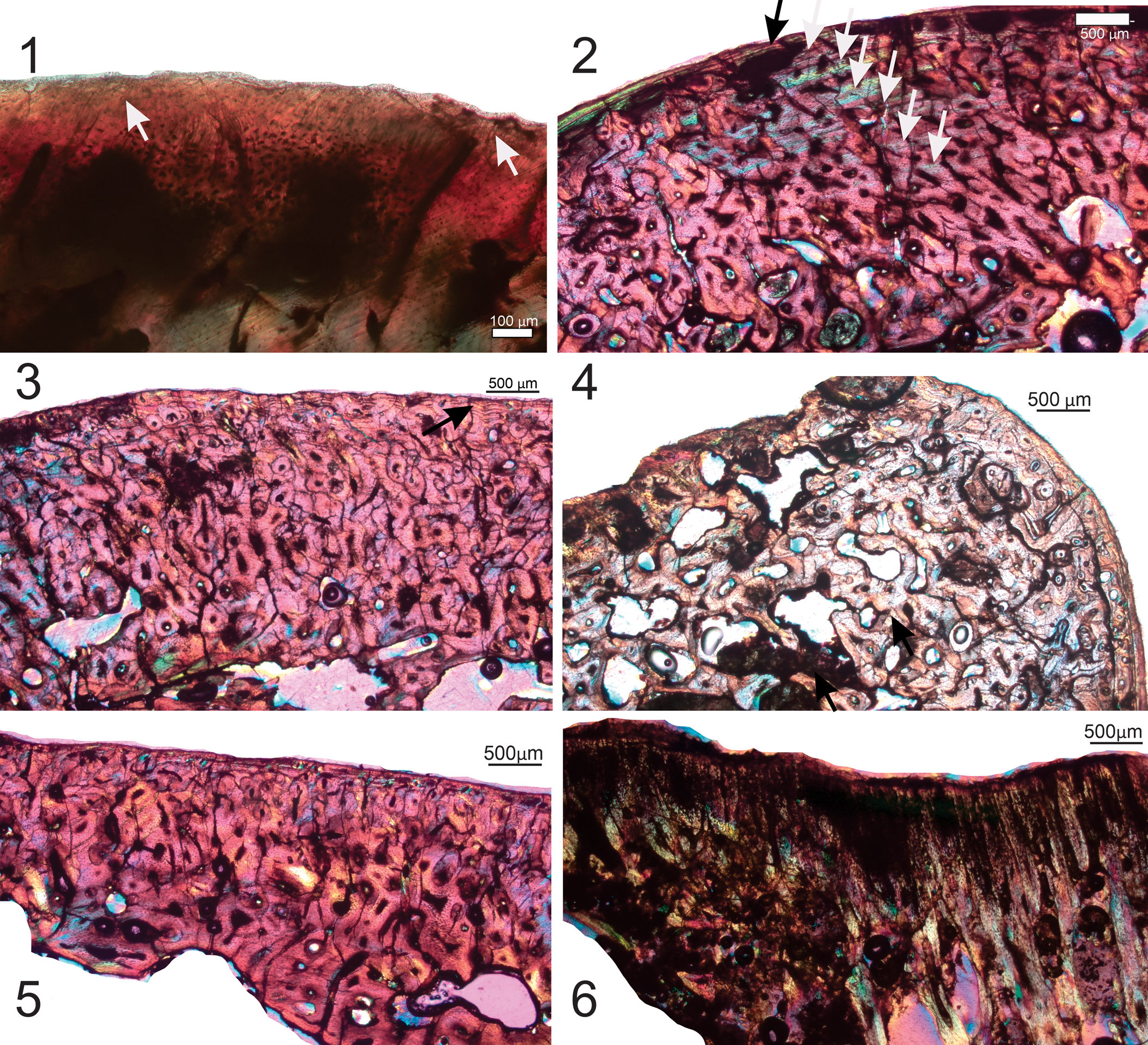
Figure 4. (1) Specimen AR21805 CII, proximal femur, arrows indicate Sharpey's fibers. (2) QM F41185, thin section AIII partial tibia, black and white arrows indicate growth marks (note the large number of erosion cavities present in the inner parts of the compacta. (3) Different part of the tibial thin section AIII from QM F41185 showing the highly remodeled cortex; the arrow indicates a group of closely spaced growth marks. (4) QM F41185, thin section AIII tibia; several erosion cavities are visible in the region of the trochanter, giving the bone a porous texture. (5) QM F41185, thin section BIII tibia; substantial remodeling in the perimedullary region leads to a narrow bone wall (note the uneven, resorptive endosteal bone margin). (6) Thin section CIII; no calcified cartilage is visible in the longitudinal section of the tibia, QM F41185. Images taken under polarized light with a one-quarter-λ compensator.
QM F41185 partial tibia
Overall, the compacta is well vascularized with a large amount of secondary osteons. However, bands of lamellar deposits of bone (annuli) are visible between the secondary osteons. At least seven annuli (often with LAGs) are observed (Fig. 4.2). At least three closely spaced growth marks are visible in the outermost region. Different parts of the cross section show different types of bone tissue. In some parts of the compacta there is a dense distribution of secondary osteons that leads to dense Haversian bone (Fig. 4.3). In the region of the trochanter there appears to be a secondary resorption of some of the secondary osteons, which leads to a more porous textured bone tissue (Fig. 4.4), and in other areas there is a large amount of remodeling in the perimedullary region that leads to a substantially narrow bone wall with several radially organized vascular canals (Fig. 4.5). No calcified cartilage is visible in the longitudinal section of the tibia (Fig. 4.6).
QM F50692 partial tibia
A thick, well-compacted outer region of the bone wall is present (Fig. 5.1), while the inner perimedullary region appears to be more cancellous (Fig. 5.2). The histology appears variable across the thin section, with different parts of the bone wall having different types of bone tissue. The outer fifth of the compacta consists predominantly of poorly vascularized primary periosteal parallel-fibered to lamellar bone tissue that is interrupted by at least 3–4 LAGs that are associated with narrow annuli. Internal to this outer tissue, the compacta is extensively remodeled, with a large number of secondary osteons present, many of which overlap (Fig. 5.1, 5.2). At higher magnification, several Sharpey's fibers (arrows) are visible near the peripheral margin of the bone wall (Fig. 5.2). In parts of the cross section, secondary reconstruction reaches up to the periosteal margin (Fig. 5.2).

Figure 5. Specimen QM F50692, partial tibia. (1) Low-magnification view of thin section BI showing thick bone wall; at least three LAGs (black arrows) are visible in outer part of the compacta. Several erosion cavities are visible in the perimedullary part of the bone wall, which give this region a cancellous texture; (2) thin section AIV; clumps of Sharpey's fibers (arrow) are visible towards the peripheral region of the bone wall; secondary remodeling extends right up to the periosteal surface in this part of the bone wall. Images taken under polarized light with a one-quarter-λ compensator.
QM F50691 partial tibia
The bone wall is highly altered by diagenesis, although there is patchy preservation of the original bone tissue (Fig. 6.1). In some areas, at least three growth marks are evident; secondary osteons are also visible (Fig. 6.2). The altered bone has the characteristics of damage caused by bacteria (e.g., Nacarino-Meneses et al., Reference Nacarino-Meneses, Chinsamy, Mayda, Kaya and Erismis2021), and there is a large amount of black infilling substance in the bone tissue.

Figure 6. QM F50691, partial tibia. The section is damaged by diagenesis. (1) Thin section AIII; at least three growth marks (white arrows) are visible in the compacta; (2) higher magnification of (1) showing the LAGs (white arrows) and a few secondary osteons; (3, 4) thin section BI showing plant material preserved inside the medullary cavity of the bone. Images (1, 2) taken under polarized light with a one-quarter-λ compensator; images (3, 4) taken under ordinary light.
Curiously, bits of plant material are preserved within the medullary cavity of this partial tibia (Fig. 6.3, 6.4). The cross section of plant material shows a central section surrounded by a few smaller cross sections. At higher magnification, the epidermis appears to be quite thick-walled, below which is a thick layer of rounded sclerenchyma, which could suggest a culm of a grass or restio. However, because grasses did not become part of the Australian flora until the Pliocene, this may represent a more recent inclusion or some other plant from the Miocene.
QM F50842a partial femur
Although this bone also shows evidence of diagenesis, the histological structure can still be deciphered (Fig. 7.1). Overall, the thin sections from this femur demonstrate how variable the histology is across the transverse section of the bone wall, as well as in different parts of the bone shaft. In a single cross section, it is evident that some parts of the bone wall are much thicker than others, whereas other areas are heavily remodeled and result in a narrow bone wall. The outer third of the bone wall appears to be composed of a more parallel-fibered to lamellar bone tissue with a mix of short radial and longitudinal vascular canals (Fig. 7.1). This tissue is interrupted by at least five GMs, several of which appear to be groups of double/multiple LAGs (Fig. 7.1). Towards the periosteal margin, there are many closely spaced LAGs (Fig. 7.1). Note that several of the vascular canals appear to cross the LAGs. Internal to this region there is a lot more secondary reconstruction, and several overlapping generations of secondary osteons are visible (Fig. 7.1, 7.2). Large erosion cavities are present nearer to the medullary cavity (Fig. 7.1). The longitudinal section of the bone shows that some endochondral ossification is still underway, since a narrow band of calcified cartilage is evident along the “articular end” of the femur (Fig. 7.3).
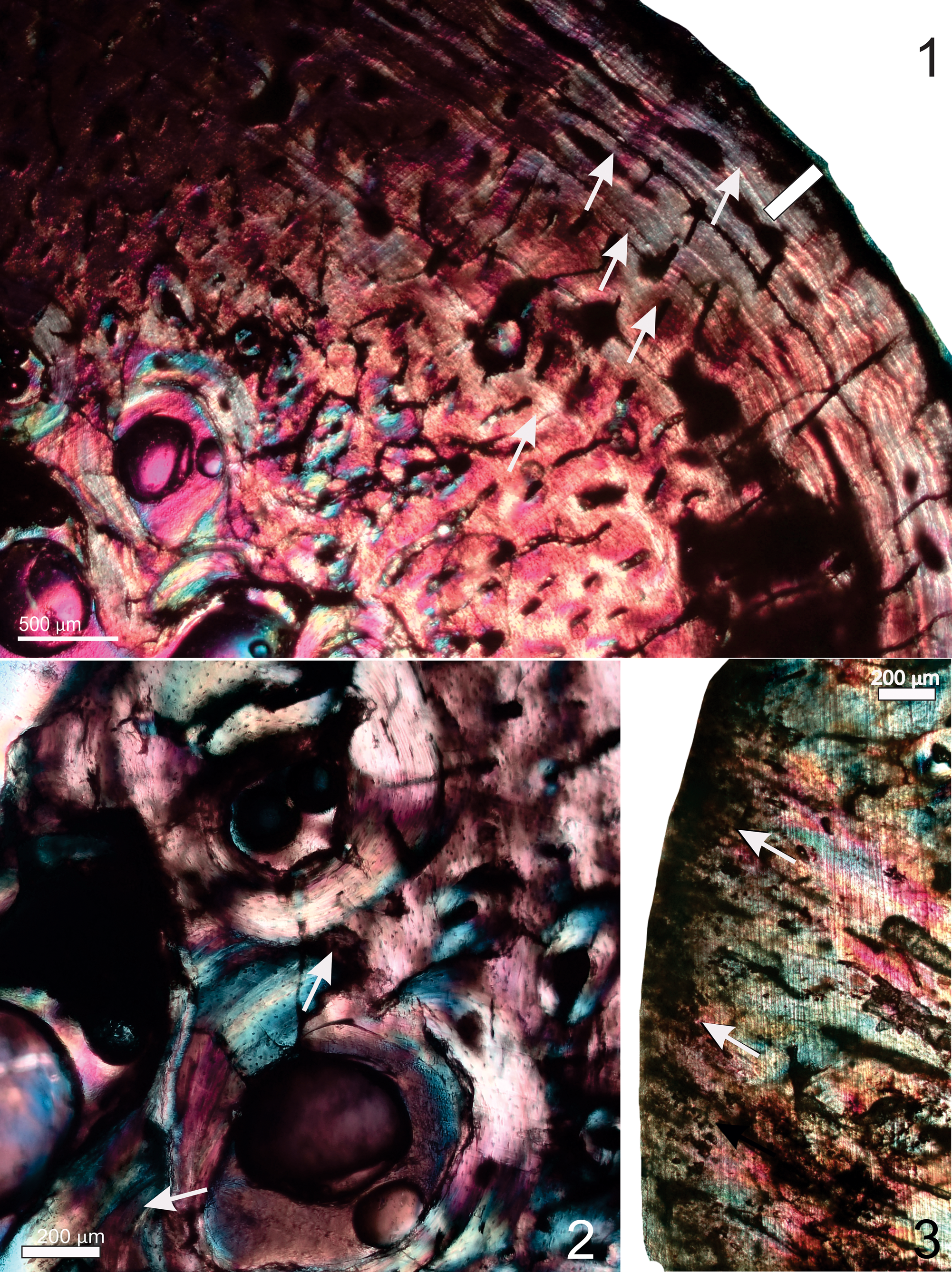
Figure 7. QM F50842a, partial femur. (1) Thin section AII; the outer third of the bone wall appears to be composed of more-lamellar bone tissue with a mix of short radial and longitudinally organized vascular canals; several growth marks interrupt the bone tissue; note that several of the vascular canals appear to cross the LAGS (arrows). In the mid-cortical region, a large amount of secondary reconstruction occurs. Many closely spaced LAGs occur in the peripheral region (white bar). (2) Thin section AII showing several large erosion cavities (white arrows) that occur nearer to the medullary cavity; overlapping generations of secondary osteons are also visible in this region. (3) Thin section DIV; the longitudinal section of the femur shows a narrow region of calcified cartilage (white arrows) at the “articular end” of the bone. Images taken under polarized light with a one-quarter-λ compensator.
Discussion
Despite substantial alteration by diagenetic factors, the histology of the femora and tibia of Nimbadon lavarackorum could be deciphered and has provided substantial information about the overall growth and biology of this diprotodontid. Juvenile individuals have a bone compacta that is composed of a mix of different types of tissues. For example, a large amount of primary bone tissue is present, although in parts of the compacta secondary reconstruction has begun but is not extensive enough to replace the original bone tissue entirely. The endosteal margin is clearly erosive in several of the juvenile specimens, and large erosion cavities are restricted to the endosteal margins. Some parts of the compacta are affected by local functional constraints. For example, attachment sites of muscles have a different histology from the rest of the compacta (Chinsamy and Warburton, Reference Chinsamy and Warburton2021).
It is well recognized that the rate at which bone forms results in different types of bone tissues (e.g., fibro-lamellar bone tissue is deposited at a faster rate as compared to parallel-fibered or lamellar bone tissue [Amprino, Reference Amprino1947; de Margerie et al., Reference de Margerie, Cubo and Castanet2002; Starck and Chinsamy, 2002]). Thus, the presence of large amounts of parallel-fibered bone as the principal primary bone tissue in most of the bones studied, including the juveniles, suggests that growth in Nimbadon occurred at a moderate rate as compared to other juvenile terrestrial mammals (including juvenile kangaroos, which tend to have large amounts of fibro-lamellar bone tissue [Chinsamy and Warburton, 2021]). However, it must be noted that although the bones studied here are juvenile, they may not be very young individuals because substantial bone remodeling and reconstruction have occurred in the bones. Thus, it is quite likely that the earliest stages of growth may have had faster deposition of bone tissue (but this is not visible in the compacta).
Based on partial femur size and morphology, specimen QM F50842a (Fig. 7) could be sub-adult, nearing adult body size. Indeed, although it is a partial specimen, its femur midshaft circumference measurement (62.5 mm) is smaller than those of adult femora (with fused epiphyses evident), which range from 65.5–68.6 mm, and there appears to be a few layers of calcified cartilage in the epiphyseal region suggesting that growth in length was still ongoing at the time of death. However, it is noteworthy that the most recently formed periosteal bone tissue in QM F50842a, as well as in the partial tibia QM F50692, is more lamellar textured, which suggests an even slower rate of bone formation than observed in the smaller (younger) long bones studied.
All the bones display growth marks (GMs) (e.g., some having at least 3–4 GMs in the peripheral region, and the partial tibia QM F41185 has at least seven GMs visible in the outer cortex; Fig. 4.2), which directly indicates that the moderate growth rates of N. lavarackorum were periodically arrested (Chinsamy-Turan, Reference Chinsamy-Turan2005). As noted for extant kangaroos (Chinsamy and Warburton, Reference Chinsamy and Warburton2021), double/multiple LAGs are visible in some individuals, indicating multiple pauses and resumption of growth in the “unfavorable season” (Castanet et al., Reference Castanet, Vieillot, Meunier, De Ricqlès and Hall1993). The close spacing of growth marks in the outer cortex of larger bones (e.g., QM F50692, Fig. 5.1; QM F50842a, Fig. 7.1) and the lamellar texture of the bone tissue indicate slow incremental growth and suggest impending skeletal maturity (although the partial femur of QM F50842a still shows some calcified cartilage [Fig. 7.3], indicating that growth in length was still occurring). Thus, as reported for the Western Grey Kangaroo (Chinsamy and Warburton, Reference Chinsamy and Warburton2021), we found that appositional growth occurred at a different rate than endochondral growth.
Among modern vertebrates, it is well recognized that the presence of growth marks (single or multiple) indicate that the growth of the animal responded to the prevailing environmental conditions, including food availability and nutritional content, as well as to endogenous physiological factors (e.g., Köhler et al., Reference Köhler, Marín-Moratalla, Jordana and Aanes2012; Angst et al., Reference Angst, Chinsamy, Steel and Hume2017). Growth marks recorded in the bones of the Western Grey Kangaroo, Macropus fuliginosus (Chinsamy and Warburton, Reference Chinsamy and Warburton2021), have been suggested to correspond to periodic decreases and stoppages in growth dynamics that occurred in response to heterothermy that the animals experienced during the harsh summers (Maloney et al., Reference Maloney, Fuller, Meyer, Kamerman, Mitchell and Mitchell2011). Similar cyclical growth patterns have been recorded in the Desert Antelope Addax nasomaculatus de Blainville, Reference de Blainville1816 (Marín-Moratalla et al., Reference Marín-Moratalla, Jordana and Köhler2013). Thus, the presence of lines of arrested growth indicates that the environment in which N. lavarackorum lived caused some physiological or nutritional stress periodically (Köhler et al., Reference Köhler, Marín-Moratalla, Jordana and Aanes2012). Based on paleoecological, biostratigraphic, and biochronological data, the paleohabitat of N. lavarackorum is interpreted to have been rainforest (Foote, Reference Foote1916; Travouillon et al., Reference Travouillon, Legendre, Archer and Hand2009; Black et al., Reference Black, Camens, Archer and Hand2012a; Zhang and Moise, Reference Zhang and Moise2016; Myers et al., Reference Myers, Black, Archer and Hand2017). Although the environmental conditions in the Middle Miocene of Australia involved less intense seasonality than today (personal communication to MA by Michael Tyler, 1995, based on fossil frog communities), it is quite likely that there may have been seasonal differences in rainfall because of monsoonal influences in the north of the continent, which might have seasonally affected the type and availability of food. The bilophodont molar morphology of N. lavarackorum suggests it was a browser, however, recent dental microwear analyses are inconsistent with obligate folivory and indicate the inclusion of a high proportion of fruit in Nimbadon's diet (Larisa De Santis, personal communication, 2022). Fruit production in the Middle Miocene rainforests of Riversleigh may have been correlated with rainfall and if so, the periodic availability of this highly nutritive food source could have contributed to the periodic increased growth rates, while the seasonal scarcity of fruit may have caused growth cessation and the formation of a LAG. Given that there is increasing evidence that several homeothermic endotherms show cyclical growth, which often is combined with seasonal decreases in body temperature and metabolic rate (e.g., Köhler et al., Reference Köhler, Marín-Moratalla, Jordana and Aanes2012; Marín-Moratalla et al., Reference Marín-Moratalla, Jordana and Köhler2013), it is likely that the growth dynamics of N. lavarackorum also may have been influenced by similar intrinsic physiological factors in conjunction with resource availability. Furthermore, although we cannot be certain, we postulate that the cyclical growth observed in this large extinct marsupial may have been linked to heterothermy, as documented for the modern Western Grey Kangaroo, Macropus fuliginosus (Maloney et al., Reference Maloney, Fuller, Meyer, Kamerman, Mitchell and Mitchell2011).
It should also be noted that xenartharans (e.g., sloths, anteaters, armadillos) all show flexible (cyclical) growth rates (Straehl et al., Reference Straehl, Scheyer, Forasiepi, MacPhee and Sánchez-Villagra2013). Interestingly, Black et al. (Reference Black, Camens, Archer and Hand2012a) found that, in terms of limb proportions, N. lavarackorum was unique among marsupials in having significantly reduced hindlimbs relative to their forelimbs, which is a feature associated in extant prosimians with suspensory climbing behaviors and/or increased pedal friction on vertical surfaces. Exploring the idea that bone compactness and structural geometry is related to lifestyle, two lineages of modern tree sloths (Choloepus and Bradypus) that are known to have slow arboreal lifestyles were examined (Girondot and Laurin, Reference Girondot and Laurin2003). They were found to have lower cortical compactness as compared to other similar-sized taxa (Nyakatura, Reference Nyakatura2012; Montañez-Rivera et al., Reference Montañez-Rivera, Nyakatura and Amson2018) and other xenarthrans, and they also show high rates of bone remodeling that appear to be maintained at high levels throughout ontogeny (Montañez-Rivera et al., Reference Montañez-Rivera, Nyakatura and Amson2018). Following these results, slow arboreal animals (e.g., tree sloths, lorisid, koala, palaeopropithecids, and Megaladapis), which all share suspensory vertical climbing and low metabolic rates, were studied to verify if they would all show low bone cortical compactness (CC) linked to their slow arboreal lifestyle (Alfieri et al., Reference Alfieri, Nyakatura and Amson2021). However, the results showed that extinct tree sloths, koalas, and lorisids all have high CC (Alfieri et al., Reference Alfieri, Nyakatura and Amson2021), following the general mammalian condition (e.g., Kolb et al., Reference Kolb, Scheyer, Veitschegger, Forasiepi, Amson, Van der Geer, Van den Hoek Ostende, Hayashi and Sánchez-Villagra2015).
Our N. lavarackorum sections were rarely complete, therefore we were unable to deduce overall CC, but based on preservation of the bone wall, it is evident that the specimens do not display low CC, and the bone wall appears to be comparable to other terrestrial vertebrates (e.g., Kolb et al., Reference Kolb, Scheyer, Veitschegger, Forasiepi, Amson, Van der Geer, Van den Hoek Ostende, Hayashi and Sánchez-Villagra2015), although clearly not as compact as fossorial animals (Montoya-Sanhueza and Chinsamy, Reference Montoya-Sanhueza and Chinsamy2017; Legendre and Botha-Brink, Reference Legendre and Botha-Brink2018; Montoya-Sanhueza et al., Reference Montoya-Sanhueza, Bennett, Oosthuizen, Dengler-Crish and Chinsamy2021b). Note that the significant amounts of cccb observed in the compacta of N. lavarackorum stems from the metaphyseal location of the sections, rather than from an adaptation for fossoriality, as observed in, for example, Ornithorhynchus (Chinsamy and Hurum, Reference Chinsamy and Hurum2006), Bathyergus (Montoya-Sanhueza and Chinsamy, Reference Montoya-Sanhueza and Chinsamy2017), Dasypus (Heck, Reference Heck, Varricchio, Gaudin, Woodward and Horner2019), and Leitheia (Kolb et al., Reference Kolb, Scheyer, Veitschegger, Forasiepi, Amson, Van der Geer, Van den Hoek Ostende, Hayashi and Sánchez-Villagra2015). Black et al. (Reference Black, Camens, Archer and Hand2012a) noted that the hindlimbs of Nimbadon are short and robust and far less specialized for climbing than the forelimbs. Furthermore, because of the lack of material, we were unable to verify if, like koalas, Nimbadon would have had higher humeral CC as compared to femora.
Interestingly, secondary osteons were not found in the cortex of koalas or in most of the marsupial bones studied (Werning, Reference Werning2013), yet in our N. lavarackorum specimens we observed a lot of secondary reconstruction throughout ontogeny. Notably though, the secondary remodeling did not reach dense Haversian proportions, but in some instances, especially in the larger individuals, overlapping generations of secondary osteons occurred. This seems to be similar to the observations made in other vombatomorphian taxa such as Vombatus ursinus Shaw, Reference Shaw1800 and Diprotodon optatum Owen, Reference Owen and Mitchell1838 (Werning, Reference Werning2013), yet, as previously noted by Werning (Reference Werning2013), it is difficult to determine whether this is a result of large body size, phylogeny, or both. Although they tend not to be extensively developed, secondary osteons have been reported in kangaroos (Chinsamy and Hurum, 2012; Chinsamy and Warburton, Reference Chinsamy and Warburton2021), but Doube et al. (2018) did not observe any secondary remodeling in a Macropus giganteus femur.
Conclusions
Our histological findings support our hypothesis that Nimbadon lavarackorum experienced cyclical growth rates, and that the number of growth rings increased through ontogeny. In addition, we found that, as expected, slower rates of bone deposition were apparent in the bone compacta as the individuals reached adult body size. The presence of seven closely spaced growth marks in the partial tibia, QM F41185, suggests that they needed at least 7–8 years to reach skeletal maturity. Indeed, given the extensive remodeling observed in the bones, it is likely that earlier growth marks may have been removed, suggesting that they may have needed even more time to reach skeletal maturity. The cyclical growth dynamics evident in the skeletal elements indicate that their growth was responsive to prevailing environmental conditions (including resources) in conjunction with intrinsic physiological factors. Bone tissue that formed during the favorable growing season appears to have been deposited at a much slower rate than in modern kangaroos. This observation suggests that N. lavarackorum grew at much slower rates, which indicates that it probably had a protracted life span to reach its large body size. Unlike koalas, N. lavarackorum has a large amount of secondary reconstruction in their bones, which suggests that they had higher demands for calcium mobilization. Because secondary remodeling is also related to biomechanical stresses during locomotion (e.g., McFarlin et al., Reference McFarlin, Terranova, Zihlman, Enlow and Bromage2008), these findings suggest that, unlike koalas, N. lavarackorum may have more actively traversed the rainforest canopy in search of fruit.
Acknowledgments
J. van der Blerk and S. Wood are thanked for assisting with preparation of thin sections at the University of Cape Town. K. Frankiewicz is thanked for some interesting discussion about the plant remains found in the medullary cavity of one of the specimens. Funding that supported this research was obtained from the National Research Foundation, South Africa, grant number, 136510, and the Australian Research Council (grant numbers DE130100467, DP170101420). We thank Queensland Parks and Wildlife Service, Environment Australia, Outback at Isa, Mount Isa City Council, Phil Creaser, and the CREATE fund at UNSW, the Rackham family, the University of New South Wales, Queensland Museum, and the Waanyi Aboriginal Community of northwestern Queensland for their ongoing support of the Riversleigh Research Project. Three reviewers are thanked for their comments, which have enhanced our manuscript.
Declaration of competing interests
The authors declare none.