Introduction
Amino acids are the fundamental building blocks of skeletal muscle and other bodily proteins. In total, there are twenty proteinogenic amino acids that when combined via peptide bonds produce proteins that are incorporated into various tissues. Nine of the twenty amino acids are deemed ‘indispensable’ or essential amino acids (EAA); the human body is unable to synthesize them endogenously in quantities sufficient to meet requirements(Reference Trumbo, Schlicker and Yates1), and they therefore must be obtained exogenously via dietary intake. The branched-chain amino acids (BCAA) represent three of the nine EAA that together account for ∼14% of the amino acids found in skeletal muscle proteins(Reference Ferrando, Williams and Stuart2). They are neutral (nonpolar and hydrophobic) amino acids and are unique in that they contain a non-linear (branched) aliphatic side chain. At the whole-body level, BCAA physiology can be divided into a tissue pool and a circulating pool. BCAA that are derived from dietary intake or liberated from protein via protein breakdown appear in the circulation. BCAA are then taken up from the circulation into body tissues (e.g. skeletal muscle) where they can be either oxidized or incorporated into proteins via protein synthesis(Reference Neinast, Murashige and Arany3). The tissue distribution of enzymes involved in BCAA catabolism and oxidation (i.e. branched chain amino transferase (BCAT) and branched chain amino acid dehydrogenase (BCKDH) complex) differs between humans and rats(Reference Hutson, Sweatt and Lanoue4,Reference Suryawan, Hawes and Harris5) . Specifically, total activity of both BCAT and BCKDH enzymes is lower in humans than rats, and distribution of BCKDH (oxidative) capacity among body tissues in humans is greatest in skeletal muscle(Reference Hutson, Sweatt and Lanoue4–Reference Brosnan and Brosnan6). In addition to serving as amino acid substrates for protein synthesis, the BCAA have long been thought to have a unique role in the regulation of skeletal muscle protein turnover. Testing of the effects of BCAA on muscle protein synthesis (MPS) and muscle protein breakdown (MPB) was initially carried out on isolated rodent muscle(Reference Buse and Reid7,Reference Fulks, Li and Goldberg8) . These early in vitro studies found that BCAA stimulated rates of MPS and reduced MPB. However, when the individual BCAA were examined, the stimulatory effect on MPS was attributed primarily to leucine (not isoleucine or valine)(Reference Buse and Reid7,Reference Buse and Weigand9) . In contrast, perfusion of isolated rodent muscle with an amino acid mixture devoid of BCAA has no effect on MPS(Reference Li and Jefferson10). In support of these findings, subsequent research has determined that, of the three BCAA, leucine appears to be a key regulator of the mechanistic target of rapamycin (mTOR)(Reference Atherton, Smith and Etheridge11), a pivotal multi-subunit complex recognized for its key role in the regulation protein synthesis and cellular growth(Reference Liu and Sabatini12). Therefore, while there are many commonalities amongst the BCAA in terms of their structure and metabolism, leucine appears to have a unique signalling role amongst the BCAA.
Given the importance of BCAA, and leucine in particular, in regulating protein turnover and mTOR signalling, it is perhaps not surprising that dietary BCAA supplements have become a commercially popular means of nutritional support to enhance the response of skeletal muscle to exercise. For example, a recent study(Reference Ruano and Teixeira13) on dietary supplement use amongst fitness club members reported that BCAA were one of the most commonly used dietary supplements, consumed by ∼37% of the sampled population. The popularity of BCAA supplements is largely predicated on the notion that they can stimulate rates of MPS and suppress rates of MPB in response to exercise, the combination of which promotes a net anabolic response in skeletal muscle. However, although the role of BCAA (leucine) as a growth-regulatory signal has been known since the 1970s(Reference Buse and Reid7,Reference Fulks, Li and Goldberg8,Reference Li and Jefferson10) , the specific effect of BCAA on skeletal muscle protein turnover (i.e. the simultaneous processes of MPS and MPB) in humans is less clear. A now seminal review by Wolfe(Reference Wolfe14) published in 2017 concluded that BCAA alone are insufficient to stimulate MPS rates in humans. However, since publication of this review, additional research has emerged providing evidence that BCAA are in fact capable of stimulating rates of MPS in humans.
The purpose of this evidence-based narrative review is to provide an update and critical appraisal of the available research literature pertaining to studies examining the effects of BCAA on MPS and MPB (i.e. muscle protein turnover), as well as associated molecular signalling responses implicated in the regulation of these processes. Emphasis is placed on studies performed in healthy humans, both at rest and in response to exercise, where the collective provision of isolated free BCAA (i.e. the provision of isoleucine, leucine and valine together as free amino acids independent of other amino acids) has been utilized within the research study design. Readers specifically interested in the role of leucine as a nutrient regulator of mTOR signalling and skeletal muscle protein turnover can refer to recent reviews on the topic(Reference Ham, Caldow and Lynch15,Reference Xu, Tan and Zhang16) .
Overview of the regulation of muscle protein turnover in response to amino acids and exercise
The purpose of this section is to provide a brief overview of the regulation of muscle protein turnover in response to amino acids and acute exercise to provide context for subsequent sections of the review examining the specific effects of BCAA on MPS, MPB and associated molecular signalling responses in humans. For more extensive reviews on the effects of protein/EAA and/or exercise on muscle protein turnover and its molecular regulation, the reader is referred to the following articles(Reference Hodson and Philp17–Reference Trommelen, Betz and van Loon21).
Muscle protein synthesis in response to amino acids and exercise
Increases in muscle mass (i.e. muscle anabolism) are determined by the balance between MPS and MPB; two ongoing, dynamic and highly regulated metabolic processes. When the rate of MPS exceeds the rate of MPB (MPS > MPB), the result is a state of positive net protein balance (NPB) and muscle protein accretion. Alternatively, when the rate of MPB exceeds the rate of MPS (MPS < MPB), NPB becomes negative, leading to loss of muscle protein. In general, the principle regulators of muscle protein turnover in adult humans are nutrient availability and exercise(Reference Atherton and Smith22). In terms of nutritional factors, dietary protein-derived amino acids are the key nutrients that support anabolic processes via their uptake and incorporation into skeletal muscle proteins via the process of MPS. The postprandial stimulation of MPS following protein ingestion is transient, lasting only a few hours(Reference Atherton, Etheridge and Watt23,Reference van Vliet, Beals and Holwerda24) , and serves to replace protein that is lost in the fasted state. Of the proteinogenic amino acids, the EAA, not the non-essential amino acids (NEAA), appear largely responsible for stimulating MPS rates in humans(Reference Børsheim, Tipton and Wolf25–Reference Volpi, Kobayashi and Sheffield-Moore29). The provision of a complete mixture of EAA in young adults stimulates MPS rates in a dose-dependent manner up to 10 g EAA(Reference Cuthbertson, Smith and Babraj30); however, older adults display ‘anabolic resistance’ which manifests as a decreased sensitivity and responsiveness of MPS to EAA intake(Reference Cuthbertson, Smith and Babraj30). As noted previously, early work in rodents(Reference Buse and Reid7,Reference Li and Jefferson10) demonstrated that the independent provision of leucine enhanced MPS to a similar extent as supplying a complete mixture of all three BCAA. Similarly, some(Reference Smith, Barua and Watt31,Reference Wilkinson, Hossain and Hill32) but not all(Reference Paulussen, Alamilla and Salvador33,Reference van Vliet, Smith and Porter34) studies in humans have shown that the independent provision of leucine is able to stimulate MPS rates. Other studies have also demonstrated that leucine supplementation of a protein-containing meal(Reference Churchward-Venne, Breen and Di Donato35–Reference Murphy, Saddler and Devries38) or leucine-enriched EAA intake(Reference Katsanos, Kobayashi and Sheffield-Moore39) can further stimulate MPS rates in humans. Stimulation of postprandial MPS rates in response to EAA ingestion in humans appears contingent upon mTOR complex 1 (mTORC1) activation since administration of the mTORC1 inhibitor rapamycin blunts the postprandial stimulation of MPS rates in response to EAA ingestion(Reference Dickinson, Fry and Drummond40).
An acute bout of resistance exercise can stimulate MPS rates for 48 h(Reference Phillips, Tipton and Aarsland41); however, NPB remains negative in the absence of exogenous amino acid provision following exercise due to a concomitant stimulation of MPB(Reference Biolo, Maggi and Williams42,Reference Biolo, Tipton and Klein43) . The consumption of protein or EAA following resistance exercise further stimulates post-exercise MPS rates as compared with resistance exercise alone, and results in a positive NPB(Reference Biolo, Maggi and Williams42,Reference Biolo, Tipton and Klein43) . Therefore, acute exercise sensitizes skeletal muscle to the anabolic effects of protein/EAA feeding(Reference Biolo, Tipton and Klein43), an effect that lasts for at least 24 h(Reference Burd, West and Moore44). In young adults, protein ingestion stimulates MPS rates in a dose-dependent manner up to ∼20 g (containing ∼10 g EAA) following resistance exercise(Reference Moore, Robinson and Fry45,Reference Witard, Jackman and Breen46) . The contraction-induced stimulation of MPS in humans is blunted in response to the mTORC1 inhibitor rapamycin(Reference Drummond, Fry and Glynn47), highlighting the importance of this pathway in regulating muscle anabolism in humans. However, studies in rodent muscle suggest that mTORC1 inhibition does not fully prevent the contraction mediated stimulation of MPS(Reference Ogasawara, Fujita and Hornberger48–Reference You, McNally and Jacobs52), suggesting mTORC1-independent mechanisms may also stimulate MPS after anabolic stimuli, potentially involving the mitogen-activated protein kinase/extracellular signal-regulated kinases 1/2 (MAPK/ERK1/2) pathway(Reference Hodson, West and Philp18). In addition to amino acid availability and exercise, reduced energy availability has a strong influence on muscle protein turnover(Reference Carbone, McClung and Pasiakos53). For example, reduced energy availability reduces both basal and postprandial MPS rates(Reference Areta, Burke and Camera54,Reference Hector, Marcotte and Churchward-Venne55) and increases whole-body amino acid oxidation(Reference Knapik, Meredith and Jones56,Reference Tsalikian, Howard and Gerich57) . However, a bout of resistance exercise performed in an energy deficit can restore MPS rates to values observed at rest in a state of energy balance(Reference Areta, Burke and Camera54). Furthermore, dietary protein ingestion after resistance exercise performed in an energy deficit can further stimulate MPS above resting MPS rates in energy balance in a dose-dependent manner(Reference Areta, Burke and Camera54).
As previously indicated, stimulation of MPS in response to anabolic stimuli is primarily regulated by mTOR, an evolutionary conserved serine/threonine kinase(Reference Bamman, Roberts and Adams58). mTOR constitutes the catalytic subunit of two structurally different multiprotein complexes known as mTORC1 and mTOR complex 2 (mTORC2) that ultimately serve two different functions(Reference Jhanwar-Uniyal, Wainwright and Mohan59). mTORC1 serves as a central hub responsible for integrating signals derived from nutrients, contractile activity (e.g. exercise) and growth factors and is a key regulator of protein synthesis and cellular growth(Reference Liu and Sabatini12). Alternatively, mTORC2 activates several pro-survival pathways and governs cytoskeletal behaviour(Reference Betz and Hall60). The kinase activity of mTORC1 and its downstream targets are dynamically regulated by protein–protein interactions and intracellular translocation and colocalization (for review see Ref.(Reference Tinline-Goodfellow, Lees and Hodson61)). Once activated, mTORC1 stimulates MPS by phosphorylating the eukaryotic initiation factor 4E-binding protein 1 (4E-BP1) and p70 S6 kinase 1 (p70S6K1). Phosphorylation of 4E-BP1 via mTORC1 results in the release of eukaryotic translation initiation factor 4E (eIF4E) from 4E-BP1 and an increase in 5′ cap-dependent translation of mRNA, while phosphorylation of p70S6K1 by mTORC1 results in the phosphorylation ribosomal protein S6 (rpS6)(Reference Hodson, West and Philp18). For example, Fujita and colleagues(Reference Fujita, Dreyer and Drummond62) reported that EAA-carbohydrate co-ingestion stimulated MPS rates concomitant with an increase in Akt(Ser473) and mTOR(Ser2448) phosphorylation, along with an increase in the phosphorylation of downstream effectors p70S6K1(Thr389) and 4E-BP1(Thr37/46), and a decrease in eukaryotic elongation factor 2(Thr56) (eEF2) phosphorylation in human skeletal muscle. For an overview of key proteins and phosphorylation events implicated in the regulation of MPS in response to amino acids, exercise, and their combination, the reader is referred to the following article(Reference Hodson, West and Philp18).
Exactly how amino acids activate mTORC1 is an area of intense research interest. In vitro studies have demonstrated that increases in amino acid availability enhance mTORC1 recruitment to the lysosome via the Rag GTPases, thereby allowing lysosomal Ras homolog enriched in brain (Rheb) to stimulate mTORC1 kinase activity(Reference Sancak, Peterson and Shaul63). In human skeletal muscle, translocation of mTORC1 to the cell periphery (i.e. sarcolemmal membrane) appears important to support its activation(Reference Korolchuk, Saiki and Lichtenberg64,Reference Hannaian, Hodson and Abou Sawan65) . In support of this notion, Hodson and colleagues(Reference Hodson, Dent and Song66) reported that protein–carbohydrate co-ingestion resulted in mTOR translocation to the cell periphery human muscle 1 h after intake, which coincided with elevated S6K1 kinase activity. Similarly, isolated leucine ingestion (2 g) has recently been demonstrated to promote mTOR translocation to the cell periphery and enhance mTOR localization with the lysosome in human skeletal muscle at 30 and 60 min post-ingestion(Reference Liu and Sabatini12). In vitro studies have identified the protein complexes GAP activity towards the Rags (GATOR1 and GATOR2), Sestrin2, cellular arginine sensor for mTORC1 (CASTOR1), and S-adenosylmethionine sensor upstream of mTORC1 (SAMTOR) as amino acid sensors (i.e. they sense amino acid sufficiency or lack thereof) that ultimately act to regulate mTORC1 activity(Reference Liu and Sabatini12). Of particular interest within the context of the present review is Sestrin2, a cytosolic leucine sensor that inhibits GATOR2, preventing lysosomal translocation of mTORC1 during leucine insufficiency. Alternatively, increased leucine availability results in binding of leucine to Sestrin2, dissociating the protein from GATOR2 to relieve mTORC1 inhibition(Reference Saxton, Knockenhauer and Wolfson67,Reference Wolfson, Chantranupong and Saxton68) . It is important to highlight that our understanding of how amino acids activate mTORC1 largely comes from in vitro studies performed in various several cell types. The stimulation of mTORC1 activity by amino acids in human skeletal muscle is still poorly understood.
MicroRNA are also emerging as potentially important regulators of anabolic process in skeletal muscle(Reference Margolis and Rivas69). MicroRNA are short (twenty to twenty-two nucleotides) non-coding RNA that recognize the 3′-untranslated regions of their target mRNA substrates and silence their expression by blocking translation or inducing transcript degradation(Reference Margolis and Rivas69). MicroRNA expression has been shown to be acutely altered in response to exercise(Reference Nielsen, Scheele and Yfanti70), and both EAA(Reference Drummond, Glynn and Fry71–Reference Margolis, McClung and Murphy73) and protein(Reference Camera, Ong and Coffey74) ingestion in human muscle. Furthermore, the expression of some microRNAs (i.e. miR-206 and miR-499) has been reported to be inversely correlated with MPS rates during exercise (i.e. increased MPS rates (%/h) are associated with reduced miR-206 and miR-499 expression)(Reference Margolis, McClung and Murphy73). While microRNA expression may be altered in response to anabolic stimuli, the specific effect of BCAA ingestion on muscle microRNA expression has not yet been explored.
Muscle protein breakdown in response to amino acids and exercise
In addition to MPS, changes in MPB play a critical role in muscle remodelling and may influence the overall anabolic response. Proteins throughout the body undergo continuous turnover, which is necessary to prevent the accumulation of damaged proteins, prevent cellular dysfunction and maintain proteostasis. Although muscle anabolism is conventionally thought to be the result of stimulation of MPS rates, it may also result from a suppression of MPB(Reference Millward, Garlick and Nnanyelugo75). A number of studies have demonstrated that an acute bout of resistance exercise stimulates MPB rates(Reference Phillips, Tipton and Aarsland41,Reference Biolo, Maggi and Williams42) , an effect that can be sustained for 24 h post-exercise(Reference Phillips, Tipton and Aarsland41). In support of these observations, a number of studies(Reference Borgenvik, Apró and Blomstrand76–Reference Yang, Jemiolo and Trappe82) have reported increases in the mRNA expression patterns of the ubiquitin ligase muscle RING-finger protein-1 (MuRF1), a known regulator of proteolysis, in the early post-exercise recovery period. In addition to exercise, some(Reference Carbone, Pasiakos and Vislocky83) but not all studies(Reference Hector, McGlory and Damas84) have demonstrated an increase in MPB rates in response to energy restriction. Theoretically, nutritional strategies that are able to suppress exercise-induced increases in MPB may contribute to a more positive NPB, and therefore facilitate the accretion of muscle mass in response to resistance exercise. While protein/EAA intake and the resulting postprandial hyperaminoacidaemia stimulate MPS rates(Reference Atherton and Smith22), amino acids can also stimulate an increase in circulating insulin concentration (i.e. hyperinsulinaemia)(Reference Floyd, Fajans and Conn85). Insulin is a powerful regulator of protein turnover in humans, primarily through its capacity to supress MPB(Reference Abdulla, Smith and Atherton86), even at low circulating concentrations (i.e., ∼15–30 mU/l)(Reference Greenhaff, Karagounis and Peirce87,Reference Wilkes, Selby and Atherton88) . The stimulation of MPB that occurs in response to resistance exercise in the postabsorptive (i.e. fasted) state(Reference Biolo, Maggi and Williams42) may be prevented when amino acids are administered after exercise(Reference Biolo, Tipton and Klein43). A common claim associated with BCAA supplements within the context of exercise is that they are ‘anti-catabolic’ (i.e. they are able to attenuate exercise-induced increases in protein breakdown), and therefore are able to better support the achievement of a positive NPB after exercise(Reference Baticci and Bozzetti89).
The breakdown of muscle proteins occurs via the coordination of several systems/pathways: the ubiquitin–proteasome pathway, autophagy-lysosome system, Ca2+-dependent calpains and the cysteine protease caspase enzymes. In the ubiquitin–proteasome pathway, proteins are tagged for breakdown by ubiquitin, leading to recognition by the 26S proteasome that digests ubiquitinated proteins to smaller peptides that are ultimately degraded to amino acids by peptidases(Reference Lecker, Goldberg and Mitch90,Reference Sandri91) . In the autophagy-lysosome system, lysosomal machinery degrades intracellular protein and organelles. This system is activated in muscle cells during catabolic conditions (e.g. disuse(Reference Brocca, Cannavino and Coletto92) and caloric restriction(Reference Wohlgemuth, Seo and Marzetti93)), and is physiologically induced by both endurance(Reference Jamart, Benoit and Raymackers94) and resistance exercise(Reference Luo, Lu and Wang95). Calpains are Ca2+-dependent cysteine proteases that target myofibrillar, cytoskeletal and sarcolemmal proteins(Reference Tipton, Hamilton and Gallagher96). Sustained increases in [Ca2+] are thought to be a mechanism that prevents excessive calpain driven proteolysis(Reference Buse and Reid7). Caspases are a family of proteases that have been proposed to play a role in the initial steps of MPB(Reference Du, Wang and Miereles97). In skeletal muscle, Forkhead box O3 (FOXO3), a transcriptional regulator of the ubiquitin ligases MuRF1 and muscle atrophy F-box (MAFbx) involved in proteasome-dependent muscle atrophy(Reference Lecker98), is linked to the expression of autophagy-related genes in vivo and in C2C12 myotubes(Reference Mammucari, Milan and Romanello99,Reference Zhao, Brault and Schild100) , and regulates both these systems(Reference Zhao, Brault and Schild100). These protein degradation systems are thought to work concurrently to regulate MPB in response to a variety of conditions including exercise and nutrition(Reference Tipton, Hamilton and Gallagher96).
Dietary BCAA requirements and general recommendations
BCAA represent ∼15–25% of the amino acids found in common food sources, with protein from milk (26%), eggs (22%) and maize (21%)(Reference Gorissen and Phillips101) representing foods particularly rich in BCAA. The current World Health Organization/Food and Agriculture Organization/United Nations University guidelines(102,Reference Elango, Ball and Pencharz103) set the total requirement for the BCAA at 85 mg kg−1 d−1 (leucine: 39 mg kg−1 d−1; isoleucine: 20 mg kg−1 d−1; valine: 26 mg kg−1 d−1) for healthy adult populations. Alternatively, the mean requirement and population-safe level (upper limit of 95% confidence interval) for total BCAA, based on data obtained in healthy sedentary young men using tracer l-[1-13C]phenylalanine and the indicator amino acid oxidation (IAAO) technique, has been reported to be substantially higher; 144 and 210 mg kg−1 d−1, respectively(Reference Riazi, Wykes and Ball104). However, the mean requirement for total BCAA is likely to be even higher amongst athletes and those who habitually engage in exercise training, as overall daily IAAO-derived recommended protein intake estimates appear to be higher in younger endurance (females: ∼1·71 g kg−1 d−1(Reference Wooding, Packer and Kato105); males: ∼1·83 g kg−1 d−1(Reference Kato, Suzuki and Bannai106)) and resistance-trained (females: ∼1·53 g kg−1 d−1(Reference Malowany, West and Williamson107); males: ∼2 g kg−1 d−1(Reference Mazzulla, Abou Sawan and Williamson108)) populations. In support of this notion, a recent IAAO study(Reference Kato, Suzuki and Bannai109) demonstrated that BCAA are the primary rate-limiting amino acids in the greater daily protein requirement of endurance-trained young men. This increased requirement may reflect the need to replace amino acids (BCAA in particular) that are oxidized during exercise(Reference Tarnopolsky110), and provide amino acid substrates to support whole-body and skeletal muscle protein remodelling(Reference Moore, Camera and Areta111). Using the IAAO technique, Kato and colleagues(Reference Kato, Suzuki and Bannai106) reported a recommended protein intake of 1·83 g kg−1 d−1 after exercise in endurance-trained young men (n = 6), corresponding to a BCAA intake of ∼396 mg kg−1 d−1. Based on the overall daily recommended protein intakes reported above derived from IAAO methodology, this would equate to a BCAA intake of ∼376 mg kg−1 d−1 for endurance-trained young females(Reference Wooding, Packer and Kato105), ∼337 mg kg−1 d−1 for resistance-trained young females(Reference Malowany, West and Williamson107), and ∼440 mg kg−1 d−1 for resistance-trained young males(Reference Mazzulla, Abou Sawan and Williamson108). Although the IAAO technique has many practical advantages as a method to determine dietary protein requirements in humans(Reference Elango, Ball and Pencharz112), there are some potential limitations of the method. For example, the IAAO method typically involves testing the response to mixture of free amino acids (not intact protein) modelled after the composition of egg protein(Reference Trommelen and van Loon113). Compared with intact protein, free amino acids result in more rapid amino acid absorption and greater postprandial plasma amino acid availability(Reference Weijzen, van Gassel and Kouw114). The IAAO method applies a repeated sip-feeding protocol (i.e. hourly protein/amino acid ingestion) that does not reflect typical food intake patterns(Reference Trommelen and van Loon113). Finally, the IAAO method does not account for the possible role of increased dietary protein intake on changes in protein breakdown(Reference Wolfe, Kim and Park115). Overall, while additional research is required to confirm these findings, individuals who regularly engage in either resistance or endurance exercise training, and who are seeking to optimize post-exercise recovery and adaptation, may benefit from higher dietary protein (and therefore BCAA intake) relative to their sedentary counterparts in order to meet elevated whole-body metabolic requirements due to training.
Branched-chain amino acid transport and availability in circulatory and intramuscular pools
Like all dietary amino acids, the BCAA are absorbed by the small intestinal epithelial cells, via discrete amino acid carriers/transporters, transported to the liver via the portal vein, and then released into systemic circulation where they can be delivered and transported into skeletal muscle(Reference Miller116,Reference Stevens117) . The large neutral amino acid (LNAA) transporter, a heterodimer composed of L-type amino acid transporter 1 (LAT1), L-type amino acid transporter 2 (LAT2) and its molecular chaperone CD98 (SLC7A5, SLC7A8 and SLC3A2, respectively) is responsible for the transport of BCAA and other large neutral amino acids(Reference Bröer118–Reference Oxender and Christensen121) across the intestinal basolateral membrane. However, the primary BCAA transporter in the gut is LAT2(Reference Bröer118–Reference Oxender and Christensen121). Following uptake across the intestinal basolateral membrane, the BCAA are transported in the portal blood to the liver, a major site of metabolism for most amino acids. Results from a number of studies(Reference Brosnan and Brosnan6,Reference Wahren, Felig and Hagenfeldt122) suggest that the BCAA largely escape first pass hepatic metabolism relative to other amino acids, and are instead heavily catabolized within skeletal muscle. This observation may relate to the low hepatic expression of the mitochondrial BCAT isozyme in humans(Reference Suryawan, Hawes and Harris5). In a now seminal study, Wahren and colleagues(Reference Wahren, Felig and Hagenfeldt122) determined that in response to protein (lean beef) ingestion in men, BCAA exceeded all other amino acids regarding their escape from the splanchnic bed, arterial concentration, and uptake by peripheral tissues (e.g. leg muscle). Specifically, it was demonstrated that, in response to a protein-rich meal, isoleucine, leucine and valine accounted for >50% of the splanchnic output of amino acids while accounting for only 20% of the protein source ingested. Therefore, ingested BCAA appear to largely escape splanchnic catabolism and become predominantly available in the circulation for uptake into peripheral tissue (i.e. skeletal muscle). The influx of BCAA into skeletal muscle is largely mediated by LAT1, which is dependent on the glutamine gradient generated by the sodium-dependent neutral amino acid transporter 2 (SNAT2)(Reference Dickinson and Rasmussen123,Reference Taylor124) .
A common observation following the provision of BCAA alone is a decline in the plasma concentration of other amino acids including methionine and the aromatic amino acids(Reference Eriksson, Hagenfeldt and Wahren125,Reference Zhang, Kobayashi and Mawatari126) . Similarly, the provision of leucine alone results in a decline in the plasma concentrations of isoleucine and valine(Reference Eriksson, Hagenfeldt and Wahren125,Reference Matsumoto, Nakamura and Matsumoto127,Reference Swendseid, Villalobos and Figueroa128) , as well as other amino acids (tyrosine, phenylalanine and methionine)(Reference Eriksson, Hagenfeldt and Wahren125). However, a similar reduction in plasma amino acid concentrations is not observed in response to the isolated provision of isoleucine or valine(Reference Eriksson, Hagenfeldt and Wahren125). For example, Alvestrand and colleagues(Reference Alvestrand, Hagenfeldt and Merli129) reported that a continuous intravenous infusion of l-leucine (300 μmol min−1) to twelve healthy females over 2·5 h decreased the concentration of most other amino acids by 17–79% in plasma, and by 17–48% in the muscle intracellular free pool, when compared with the other BCAA and the aromatic amino acids. This reduction in the plasma and intramuscular concentration of select amino acids has been taken to suggest that provision of isolated BCAA (or leucine) suppresses protein breakdown (and therefore the rate at which protein-bound amino acids are released into the intracellular free pool and circulation), and/or stimulates protein synthesis(Reference Nair and Short130).
The effect of branched-chain amino acids on muscle protein synthesis and associated molecular signalling in humans
Protein synthesis is an extremely energy- and resource-intensive process in growing cells(Reference Buttgereit and Brand131). The effects of BCAA on MPS, MPB and associated molecular signalling responses may be influenced by the nutritional state of the participant when BCAA are administered, and differ depending on whether BCAA are ingested individually (e.g. leucine intake alone) or collectively (e.g. isoleucine, leucine and valine) or are co-ingested with other amino acids (e.g. as part of a complete protein or BCAA-enriched protein supplement)(Reference Tipton132). As the current review is primarily focused on studies evaluating the effects of the provision of a complete mixture of isolated BCAA, the studies discussed in this review have provided BCAA as free amino acids. It is important to highlight that the included studies vary in that some have provided BCAA via intravenous infusion(Reference Everman, Meyer and Tran133–Reference Liu, Jahn and Long136) or oral consumption(Reference Ferrando, Williams and Stuart2,Reference Churchward-Venne, Breen and Di Donato35,Reference Borgenvik, Apró and Blomstrand76,Reference Fuchs, Hermans and Holwerda137–Reference Monteyne, Coelho and Porter145) , studied participants at rest or the response in non-exercised (i.e. rested) muscle(Reference Ferrando, Williams and Stuart2,Reference Churchward-Venne, Breen and Di Donato35,Reference Borgenvik, Apró and Blomstrand76,Reference Everman, Meyer and Tran133–Reference Fuchs, Hermans and Holwerda137,Reference Apró and Blomstrand139,Reference Monteyne, Coelho and Porter145) , studied participants under post-exercise conditions(Reference Churchward-Venne, Breen and Di Donato35,Reference Borgenvik, Apró and Blomstrand76,Reference Jackman, Witard and Philp138–Reference Monteyne, Coelho and Porter145) , studied younger adults(Reference Ferrando, Williams and Stuart2,Reference Churchward-Venne, Breen and Di Donato35,Reference Borgenvik, Apró and Blomstrand76,Reference Everman, Meyer and Tran133–Reference Liu, Jahn and Long136,Reference Jackman, Witard and Philp138–Reference Monteyne, Coelho and Porter145) and studied older adults(Reference Fuchs, Hermans and Holwerda137). The included studies also vary in the BCAA dose and amount of energy provided, the corresponding ratio of isoleucine, leucine and valine within a given dose, nature of the control/comparator treatment(s), and timing of sample collection. All these factors may have implications for the study results obtained and any subsequent conclusions drawn. A detailed overview of studies in this review examining the effects of BCAA on MPS, MPB and/or associated molecular signalling responses in humans is presented in Supplementary Table 1.
Studies performed under resting conditions
A purported benefit of BCAA supplements is that they stimulate MPS rates and promote a net anabolic effect in muscle, particularly when coupled with resistance exercise. However, this claim remains a contentious issue as only a limited number of studies(Reference Everman, Meyer and Tran133–Reference Jackman, Witard and Philp138,Reference Jackman, Wallis and Yu141,Reference Moberg, Apró and Ekblom144) have been performed in humans addressing the effect of a complete mixture of isolated BCAA on MPS rates. To the best of our knowledge, Louard and colleagues(Reference Louard, Barrett and Gelfand134) performed the first investigation in humans (men and women aged 18–34 years) examining the effects of isolated BCAA on whole-body and MPS in the overnight post-absorptive state under resting (i.e. non-exercise) conditions. In a parallel-group design, the BCAA were provided to one group of participants via intravenous infusion, and their effects were compared against another group of participants who were infused with saline as a control. The researchers found that infusion of BCAA for 3 h led to a marked increase in indices of whole-body protein synthesis (i.e. based on measures of non-oxidative leucine disposal). At the muscle level, BCAA infusion led to different amino acid kinetic responses based on arteriovenous (A-V) exchange measurements across the forearm depending on the amino acid tracer examined (i.e., l-[ring-2,6-3H]-phenylalanine versus l-[1-14C]-leucine). Specifically, the phenylalanine tracer showed no increase in indices of MPS (i.e. rate of disappearance (Rd) (nmol min−1 100 ml−1)) in response to BCAA infusion, while the leucine tracer showed a marked increase. Based on the phenylalanine data, the authors concluded that BCAA do not stimulate MPS. A subsequent similar parallel group study by the same group(Reference Louard, Barrett and Gelfand135) investigated the effects of a more prolonged (16 h) overnight intravenous infusion of BCAA in the overnight fasted state on whole-body and skeletal muscle amino acid kinetics in both men and women (18–34 years) using the same methodology. The results were compared against a group who received a 4 h systemic intravenous infusion of saline. Similar results were obtained at the whole-body and muscle level. Once again, infusion of BCAA did not stimulate rates of MPS based on A-V exchange measurements across the forearm using isotope-labelled phenylalanine, suggesting the lack of a stimulatory effect on MPS rates in their earlier study(Reference Louard, Barrett and Gelfand135) was not due to an insufficient duration of BCAA infusion.
In a crossover study design, Liu and colleagues(Reference Liu, Jahn and Long136) evaluated the effects of isolated BCAA at rest, administered via intravenous infusion in the overnight fasted state, on whole-body phenylalanine flux (rate of appearance (Ra)), forearm phenylalanine kinetics and the phosphorylation of eIF-4E-BP1 (β + γ/α + β + γ ratio) and p70S6K (β + γ/α + β + γ ratio) with and without dexamethasone treatment. In the absence of dexamethasone treatment, infusion of BCAA improved forearm phenylalanine net balance when assessed at 6 h post infusion. However, while BCAA infusion increased the phosphorylation status of both eIF4E-BP1 and p70S6K, it did not increase indices of MPS (i.e. phenylalanine Rd (nmol min−1 100 ml−1)). The authors concluded that BCAA act directly as nutrient signals in human skeletal muscle to activate mRNA translation and potentiate protein synthesis(Reference Liu, Jahn and Long136).
In 2016, Everman and colleagues(Reference Everman, Meyer and Tran133) examined healthy young males and females in the overnight fasted state before and after insulin infusion to determine whether insulin stimulates MPS in relation to the availability of BCAA alone. No differences in MPS rates were found in response to BCAA versus saline infusion under both basal and insulin-stimulated conditions when MPS was assessed using the gold-standard precursor–product approach (i.e., l-[ring-2H5]-phenylalanine incorporation into muscle protein sampled via needle biopsy). Specifically, mean (SD) plasma concentrations of BCAA were 282 ± 40 and 310 ± 41 μmol/l during basal and insulin infusion periods in the saline condition, and 1059 ± 140 and 933 ± 91 μmol/l during basal and insulin infusion periods in the BCAA condition. The authors concluded that insulin does not stimulate MPS rates in the presence of increased circulating levels of plasma BCAA alone.
While the provision of BCAA via intravenous administration does provide valuable information on their effect on muscle protein turnover, outside of a controlled laboratory setting, this is an uncommon and highly impractical means of administration. Ferrando and colleagues(Reference Ferrando, Williams and Stuart2) assessed the effects of orally ingested BCAA (5·2 g leucine, 2·6 g isoleucine, 3·2 g valine), co-ingested with carbohydrate (50 g) in the overnight fasted state, against an iso-nitrogenous and iso-caloric carbohydrate–EAA drink (4·0 g threonine, 3·8 g histidine, 3·2 g methionine) on leg A-V phenylalanine balance and postprandial MPS rates in young men using the precursor–product approach. Examination of leg phenylalanine kinetics via A-V balance revealed no stimulatory effect of BCAA on leg protein synthesis. While not statistically significant, BCAA stimulated MPS rates (i.e. fractional synthesis rate (FSR)) from 0·047 ± 0·002 during basal conditions to 0·093 ± 0·020 %/h in the postprandial period, while the EAA solution stimulated MPS rates from 0·059 ± 0·017 to 0·073 ± 0·015 %/h(Reference Ferrando, Williams and Stuart2).
Recently, Fuchs and colleagues(Reference Fuchs, Hermans and Holwerda137) compared the impact of ingesting 6 g BCAA, 6 g branched-chain ketoacids and 30 g milk protein (containing 6 g BCAA) at rest in the overnight fasted state on postprandial myofibrillar protein synthesis (MyoPS) rates in older men (71 ± 1 years) using the precursor–product approach. It was reported that ingestion of BCAA stimulated (from 0·022 ± 0·002 %/h to 0·044 ± 0·004 %/h) postprandial MyoPS rates comparable to that elicited in response to ingestion of 30 g milk protein (from 0·020 ± 0·002 %/h to 0·042 ± 0·004 %/h) during the early (0–2 h) postprandial period. However, whereas the ingestion of 30 g of milk protein was able to sustain elevated MyoPS rates during the late (2–5 h) postprandial period (0·039 ± 0·004 %/h), the stimulation of MyoPS rates following isolated BCAA intake was short-lived, and not sustained during the late postprandial period (0·024 ± 0·005 %/h)(Reference Fuchs, Hermans and Holwerda137) (Fig. 1). Interestingly, branched-chain ketoacid administration also elicited a transient stimulation of MyoPS rates that was similar to that achieved with BCAA. This work clearly demonstrates that while isolated BCAA (and branched-chain ketoacid) intake can stimulate postprandial MyoPS rates in older adults, the response is transient, likely due to insufficient availability of the other amino acids required as substrate to yield a sustained stimulation of MyoPS rates(Reference Wolfe14).
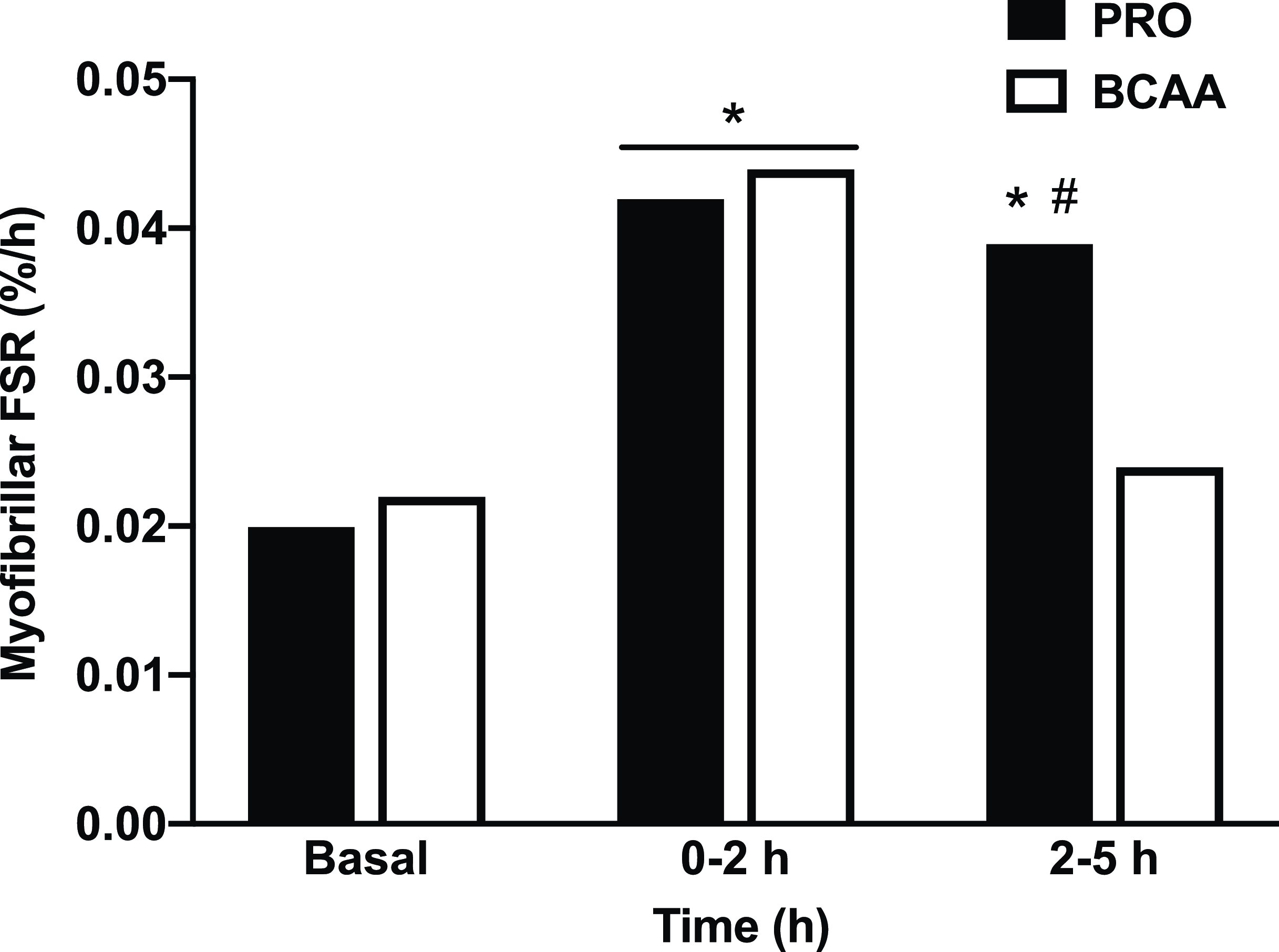
Fig. 1. Myofibrillar protein fractional synthesis rate (FSR; %/h) during the fasted (basal) state and over the early (0–2 h), and late (2–5 h) postprandial period following the ingestion of 30 g milk protein (PRO; complete source of protein containing ∼6 g BCAA, of which 2·64 g was leucine) or 6 g branched-chain amino acids (BCAA; 3 g leucine, 1·5 g isoleucine, 1·5 g valine) in healthy older males. Values represent means. *Significantly different from basal; #significantly different from BCAA at the same timepoint. Adapted from Fuchs CJ, et al. (2019)(Reference Fuchs, Hermans and Holwerda137).
Studies performed with resistance exercise
In 2017, Jackman and colleagues(Reference Jackman, Witard and Philp138) reported that the oral intake of BCAA (BCAA: 5·6 g; 1·4 g isoleucine; 2·6 g leucine; 1·6 g valine) following an acute bout of resistance exercise performed 3 h following a standardized breakfast, stimulated 22% greater MyoPS rates in young men over 4 h post-exercise recovery, when compared with an energy-matched carbohydrate control. In this study, MyoPS rates were determined via the precursor–product approach. The greater rates of MyoPS following BCAA intake were accompanied by enhanced phosphorylation of Akt(Ser473), PRAS40(Thr246) and p70S6K1(Thr389) at 1 h after consumption versus baseline only in the BCAA trial(Reference Jackman, Witard and Philp138). These findings are in alignment with those of Liu and colleagues(Reference Liu, Jahn and Long136) who reported an increase in the phosphorylation p70S6K (β + γ/α + β + γ ratio) in response to BCAA infusion at rest. However, Jackman and colleagues(Reference Jackman, Witard and Philp138) noted that the stimulation of MyoPS rates in response to BCAA intake following resistance exercise was ∼50% less than the previously reported MyoPS response to a dose of whey protein containing similar amounts of BCAA(Reference Witard, Jackman and Breen46,Reference Churchward-Venne, Burd and Mitchell146) . These results suggest that, while isolated BCAA ingestion can result in greater MyoPS rates after an acute bout of resistance exercise as compared with an energy-matched carbohydrate control, ingestion of the full complement of EAA via high-quality dietary protein (e.g. whey protein) intake may be required to optimally stimulate MyoPS rates after resistance exercise (Fig. 2).
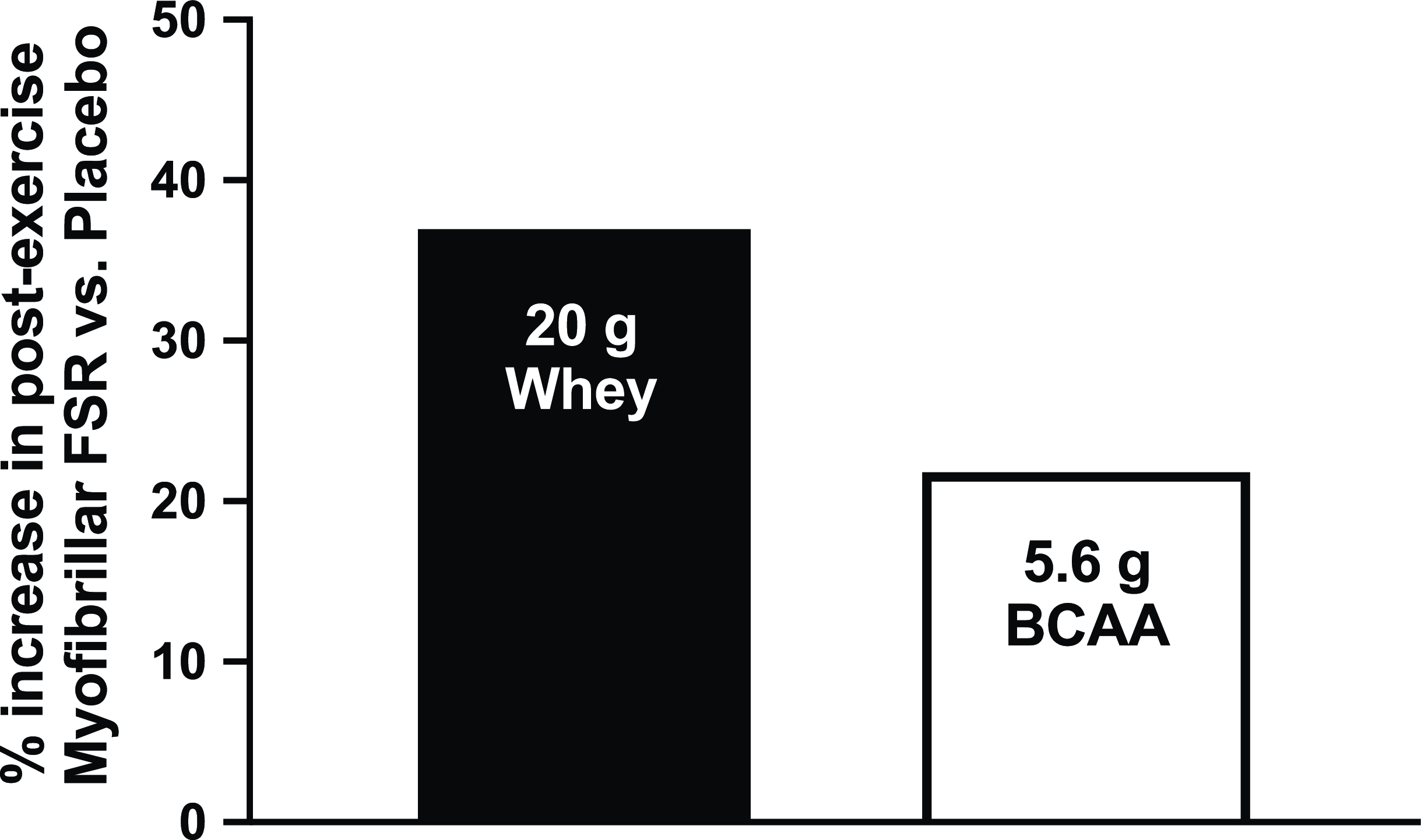
Fig. 2. Per cent increase in post-exercise myofibrillar protein fractional synthesis rate (FSR) versus placebo after ingestion of 20 g whey protein isolate (containing 10 g of EAA and 4·8 g of BCAA) or 5·6 g BCAA (containing 2·6 g leucine, 1·4 g isoleucine, 1·6 g valine). Post-exercise ingestion of 20 g whey protein isolate yields a 37% greater post-exercise myofibrillar protein FSR compared with 0 g whey protein isolate ingestion (placebo). Post-exercise ingestion of 5·6 g BCAA yields a 22% greater post-exercise myofibrillar protein FSR compared with 0 g BCAA ingestion (placebo). Adapted from Jackman et al. (2017)(Reference Jackman, Witard and Philp138) and Witard et al. (2014)(Reference Witard, Jackman and Breen46).
More recently, Jackman and colleagues(Reference Jackman, Wallis and Yu141) evaluated the effects of co-ingestion of BCAA (BCAA: 6·1 g; 1·4 g isoleucine; 2·8 g leucine; 1·9 g valine) with carbohydrate (30·6 g) on MyoPS rates after an acute bout of resistance exercise performed 3 h following a standardized breakfast in trained young men. BCAA co-ingestion with carbohydrate stimulated ∼15% greater MyoPS rates over 4 h post-exercise recovery when compared with an energy-matched carbohydrate control. In qualitative terms, this 15% increase is similar to the 22% increase in post-exercise MyoPS rates with BCAA the authors reported previously(Reference Jackman, Witard and Philp138), and again suggest that isolated BCAA intake may not result in an optimal muscle anabolic environment following resistance exercise.
In partial contrast to the studies from Jackman and colleagues(Reference Jackman, Witard and Philp138,Reference Jackman, Wallis and Yu141) , Moberg and colleagues(Reference Moberg, Apró and Ekblom144) reported no differences between leucine (50 mg/kg), BCAA (110 mg/kg: 25% l-isoleucine, 45% l-leucine and 30% l-valine), EAA (290 mg/kg) and placebo (flavoured water) ingestion on postprandial MPS rates following acute resistance exercise. Although there were no differences between treatments on post-exercise MPS rates, p70S6K1 activity (pmol min−1 mg−1) increased above resting values in all four trials, such that placebo < leucine < BCAA < EAA when assessed 90 min following exercise. Furthermore, p70S6K1 activity after 180 min of recovery remained ∼60–95% higher in the BCAA and EAA trials versus the placebo and leucine trial(Reference Moberg, Apró and Ekblom144). Similar findings were also observed when evaluating the phosphorylation status of 4E-BP1(Thr46) and 4E-BP1(Ser65) at 90 and 180 min post-exercise. Taken together, these results suggest that a mixture of EAA promotes early signalling (90 min post-exercise) responses associated with translation initiation to a greater extent than BCAA; however, differences in signalling responses between BCAA and EAA become less apparent during the later stages (180 min) of post-exercise recovery.
A number of other studies have evaluated changes in molecular signalling implicated in the regulation of MPS in response to isolated BCAA intake in human muscle during recovery after exercise(Reference Borgenvik, Apró and Blomstrand76,Reference Apró and Blomstrand139,Reference Ferreira, Li and Cooke140,Reference Karlsson, Nilsson and Nilsson142,Reference Lysenko, Vepkhvadze and Lednev143) (see Supplementary Table 1 for details). Collectively, these studies support the notion that BCAA intake may further enhance p70S6K1(Thr389)(Reference Borgenvik, Apró and Blomstrand76,Reference Apró and Blomstrand139,Reference Karlsson, Nilsson and Nilsson142) and rpS6(Ser235/236)(Reference Apró and Blomstrand139,Reference Karlsson, Nilsson and Nilsson142) , but not eEF2(Thr56)(Reference Apró and Blomstrand139,Reference Moberg, Apró and Ekblom144) phosphorylation during the early recovery period after resistance exercise. Alternatively, the effect of BCAA intake on mTOR(Ser2448) phosphorylation is less clear, with one study reporting an increase(Reference Moberg, Apró and Ekblom144), and other studies reporting no difference(Reference Borgenvik, Apró and Blomstrand76,Reference Apró and Blomstrand139,Reference Ferreira, Li and Cooke140) versus placebo ingestion during recovery after resistance exercise. Overall, BCAA intake appears to further enhance the phosphorylation status of some proteins within the mTORC1 pathway involved in the regulation of translation initiation of MPS in humans.
Fortification of dietary protein with a complete mixture of BCAA
Some research has evaluated the effects of supplementing various doses of protein with a complete mixture of BCAA on MyoPS rates both at rest and following resistance exercise in healthy adults(Reference Churchward-Venne, Breen and Di Donato35,Reference Monteyne, Coelho and Porter145,Reference Engelen, Rutten and De Castro147) . For example, Churchward-Venne and colleagues(Reference Churchward-Venne, Breen and Di Donato35) evaluated the effects of supplementing a ‘sub-optimal’ dose (6·25 g) of whey protein with different doses of leucine, as well as a mixture of BCAA (total BCAA intake: 7·73 g), compared with a more ‘optimal’ 25 g dose of whey protein. Ingestion of 6·25 g whey protein supplemented with BCAA in the overnight fasted state did not stimulate postprandial MyoPS rates, at rest or following acute resistance exercise, as effectively as 25 g whey protein. Similarly, Monteyne and colleagues(Reference Monteyne, Coelho and Porter145) reported that supplementing a lower dose (18·7 g protein) of mycoprotein with BCAA (total: 2·5 g leucine, 1·5 g isoleucine and 1·9 g valine) failed to stimulate MPS rates, both at rest and following resistance exercise, as effectively as a larger dose (35·1 g protein) of mycoprotein matched for BCAA content. Finally, although MPS rates were not assessed, Engelen and colleagues(Reference Engelen, Rutten and De Castro147) reported that adding BCAA to a soy protein meal did not alter whole-body protein synthesis or net balance when compared with a soy protein meal without BCAA fortification in a group of healthy older men studied under resting conditions. However, adding BCAA to a soy protein meal further stimulated whole-body protein synthesis in older men with COPD. While the addition of a mixture of BCAA to protein (i.e. to whey or mycoprotein) represents a different supplementation model/strategy than isolated ingestion of all three BCAA without other amino acids, these results further support the notion that ingestion of an ample amount of high-quality protein containing sufficient quantities of the full complement of amino acids appears necessary to robustly stimulate postprandial MPS rates.
Overall, some(Reference Fuchs, Hermans and Holwerda137,Reference Jackman, Witard and Philp138,Reference Jackman, Wallis and Yu141) but not all(Reference Ferrando, Williams and Stuart2,Reference Everman, Meyer and Tran133,Reference Moberg, Apró and Ekblom144) studies applying the gold-standard precursor–product approach to assess MyoPS or MPS rates following oral (as opposed to intravenous) BCAA intake have demonstrated that BCAA can stimulate MPS rates in humans, both at rest(Reference Fuchs, Hermans and Holwerda137) and following resistance exercise(Reference Jackman, Witard and Philp138,Reference Jackman, Wallis and Yu141) . However, the stimulation of postprandial MPS rates following isolated BCAA intake is transient and sub-optimal when compared with the MPS response achieved following the ingestion of a complete protein (e.g. whey) matched for BCAA intake but providing the full complement of amino acids. Furthermore, the large majority of studies to date examining the effects of BCAA on MPS rates and associated molecular signalling responses have lacked an iso-caloric and/or iso-nitrogenous EAA control (Supplementary Table 1), making it difficult to determine whether the BCAA are more anabolic than other EAA in human muscle. Therefore, ingestion of a sufficient dose of high-quality dietary protein containing a complete mixture of amino acids represents a superior option to isolated BCAA intake for stimulating MPS rates in human muscle.
The effect of branched-chain amino acids on muscle protein breakdown and associated molecular signalling in humans
Studies examining the response of human skeletal muscle protein turnover to exercise and nutritional stimuli have focused predominantly on the response of MPS(Reference Tipton, Hamilton and Gallagher96,Reference Pasiakos and Carbone148) , with much less information available on the response of MPB. This is largely due to the technical challenges associated with obtaining dynamic measures of MPB(Reference Tipton, Hamilton and Gallagher96,Reference Pasiakos and Carbone148) . As discussed, MPB is a determinant of NPB, and therefore can influence the overall anabolic response of muscle to nutrition and exercise interventions. The process of MPB also provides amino acid substrate for protein synthesis in other bodily organs and tissues, supports the repair, remodelling and synthesis of muscle proteins, and provides amino acids for hepatic gluconeogenesis(Reference Pasiakos and Carbone148). A common claim pertaining to BCAA is that they are capable of suppressing rates of MPB during exercise and therefore are ‘anti-catabolic’. In partial support of this notion, a number of early in vitro studies in rodent skeletal muscle tissue reported that BCAA reduced protein breakdown (for review see Ref.(Reference May and Buse149)). However, only a limited number of studies to date have examined the effects of a complete mixture of BCAA on indices of MPB in vivo in humans, and none has examined the effects of BCAA on MPB rates in response to resistance exercise.
The aforementioned studies by Louard and colleagues examining the effects of BCAA on whole-body and muscle protein metabolic responses(Reference Louard, Barrett and Gelfand134,Reference Louard, Barrett and Gelfand135) included measurements of whole-body and MPB (derived from A-V exchange measurements across the forearm using isotopically labelled amino acids). In response to a 3 h infusion of BCAA, phenylalanine flux (Ra) was reduced by 22%, indicative of a reduction in whole-body protein breakdown. Similar results were obtained from the phenylalanine tracer exchange data across the forearm; that is, muscle phenylalanine appearance (Ra) from protein breakdown was reduced in response to BCAA infusion(Reference Louard, Barrett and Gelfand134). This reduction in protein breakdown was unlikely due to a BCAA-mediated increase in insulin availability, as BCAA infusion did not alter insulin concentrations. A similar ∼37% reduction in whole-body phenylalanine flux (Ra) and decrease in forearm protein breakdown (i.e. phenylalanine Ra) was observed by the same researchers in response to a prolonged (16 h) overnight intravenous infusion of BCAA(Reference Louard, Barrett and Gelfand135). Alternatively, the aforementioned studies by Ferrando and colleagues(Reference Ferrando, Williams and Stuart2) and Liu and colleagues(Reference Liu, Jahn and Long136) failed to detect a decrease in indices of MPB in response to BCAA administration based on A-V exchange measurements across the leg and forearm respectively, using isotopically labelled phenylalanine. Nonetheless, BCAA reduced whole-body phenylalanine flux (Ra) in both studies(Reference Ferrando, Williams and Stuart2,Reference Liu, Jahn and Long136) , suggesting a suppression of whole-body protein breakdown. Other studies have also provided support for the notion that BCAA may attenuate whole-body protein breakdown, as BCAA have been reported to reduce whole-body phenylalanine flux (Ra) during the initial hours of post-exercise recovery(Reference Jackman, Witard and Philp138,Reference Jackman, Wallis and Yu141) . While BCAA may attenuate both whole-body and MPB, the molecular mechanisms underpinning the anti-proteolytic effects of BCAA are unclear.
There is some evidence(Reference Borgenvik, Apró and Blomstrand76,Reference Lysenko, Vepkhvadze and Lednev143) that BCAA reduce the expression (mRNA and/or protein) of the ubiquitin ligases MuRF-1 and MAFbx in human skeletal muscle. For example, Borgenvik and colleagues(Reference Borgenvik, Apró and Blomstrand76) reported that BCAA (85 mg BCAA/kg body weight: 45% leucine, 30% valine and 25% isoleucine) reduced MAFbx mRNA expression in both resting and resistance exercised human muscle, and attenuated the increase in MuRF-1 total protein expression compared with a placebo in young adults. Similarly, Lysenko and colleagues(Reference Lysenko, Vepkhvadze and Lednev143) reported that BCAA supplementation (0·1 g BCAA/kg body weight: 50% leucine, 25% isoleucine and 25% valine) reduced the mRNA expression of MAFbx (Atrogin-1) and MuRF-1 at select timepoints during recovery from an acute bout of endurance exercise in young endurance-trained athletes(Reference Lysenko, Vepkhvadze and Lednev143). Overall, a number of studies have demonstrated that a mixture of BCAA can attenuate both whole-body(Reference Ferrando, Williams and Stuart2,Reference Louard, Barrett and Gelfand134–Reference Liu, Jahn and Long136,Reference Jackman, Witard and Philp138,Reference Jackman, Wallis and Yu141) as well as indices of muscle(Reference Louard, Barrett and Gelfand134,Reference Louard, Barrett and Gelfand135) protein breakdown, and reduce the mRNA expression of the ubiquitin ligases MAFbx and MuRF-1(Reference Borgenvik, Apró and Blomstrand76,Reference Lysenko, Vepkhvadze and Lednev143) . However, no studies to date have assessed whether BCAA intake can suppress the elevation in MPB rates that occurs during the post-exercise recovery period in response to resistance exercise(Reference Phillips, Tipton and Aarsland41,Reference Phillips, Tipton and Ferrando150) . Given that insulin, even at relatively low circulating concentrations (i.e. ∼15–30 mU/l), can strongly suppress muscle proteolysis(Reference Greenhaff, Karagounis and Peirce87,Reference Wilkes, Selby and Atherton88) , mixed macronutrient intake (e.g. intake of both carbohydrate and protein) would also be expected to suppress MPB, and as discussed, better support the stimulation of MPS rates in human muscle.
Conclusion
The aim of this narrative review was to critically appraise the available research literature from studies performed in humans examining the effects of BCAA on MPS, MPB and associated molecular signalling pathway responses. Emphasis was placed on results from studies implementing a supplementation model involving isolated intake of all three BCAA (i.e. the provision of leucine, isoleucine and valine together as free amino acids, independent of other amino acids), rather than on the effects of leucine alone. Recent studies have demonstrated that isolated intake of BCAA can stimulate postprandial MyoPS rates above basal conditions at rest(Reference Fuchs, Hermans and Holwerda137), and result in greater MyoPS rates as compared with energy-matched carbohydrate intake during recovery following resistance exercise(Reference Jackman, Witard and Philp138,Reference Jackman, Wallis and Yu141) . However, the stimulatory effect of BCAA intake on postprandial MyoPS rates at rest and after resistance exercise is transient and/or reduced compared to the MyoPS response achieved following the ingestion of 25–30 g of a high-quality complete protein source containing all EAA(Reference Fuchs, Hermans and Holwerda137,Reference Jackman, Witard and Philp138) . Along these lines, isolated BCAA intake can enhance the activation/phosphorylation status of some signalling proteins within the mTORC1 pathway known to regulate translation initiation after exercise(Reference Borgenvik, Apró and Blomstrand76,Reference Liu, Jahn and Long136,Reference Jackman, Witard and Philp138,Reference Apró and Blomstrand139,Reference Karlsson, Nilsson and Nilsson142,Reference Moberg, Apró and Ekblom144) . However, a complete mixture of all EAA (as would be contained within high-quality dietary protein) appears to stimulate translation initiation signalling in skeletal muscle more effectively than just the BCAA(Reference Moberg, Apró and Ekblom144).
There is currently a paucity of data on the nutritional regulation of MPB rates following exercise. To our knowledge, no studies to date have examined the effects of isolated BCAA intake on MPB rates following resistance exercise. Nonetheless, BCAA appear capable of suppressing both whole-body(Reference Ferrando, Williams and Stuart2,Reference Louard, Barrett and Gelfand134,Reference Louard, Barrett and Gelfand135) and muscle (limb)(Reference Louard, Barrett and Gelfand134,Reference Louard, Barrett and Gelfand135) , protein breakdown during resting (i.e. non-exercised) conditions, and whole-body protein breakdown during recovery after resistance exercise(Reference Jackman, Witard and Philp138,Reference Jackman, Wallis and Yu141) . The molecular mechanisms responsible for this effect are unclear but may partially relate to a BCAA-mediated reduction in the expression of the ubiquitin ligases MAFbx and MuRF-1(Reference Borgenvik, Apró and Blomstrand76,Reference Lysenko, Vepkhvadze and Lednev143) . While the suppression of MPB after exercise may contribute to a more anabolic environment, it is not clear that the suppression of whole-body and/or MPB after exercise is desirable for enhanced muscle hypertrophy, muscle remodelling and adaptation to training, and/or post-exercise exercise recovery processes(Reference Tipton, Hamilton and Gallagher96,Reference Tipton132) . Overall, based on the currently available evidence, orally ingested BCAA supplements activate molecular signalling involved in translation initiation, and can reduce the expression of the ubiquitin ligases MAFbx and MuRF-1. Along these lines, BCAA appear to reduce indices of MPB at rest, and whole-body protein breakdown both at rest and during recovery from resistance exercise; however, their effect on resistance exercise-induced MPB rates has not been evaluated. Although some studies have demonstrated that BCAA can transiently stimulate MyoPS rates, most studies to date have lacked an iso-caloric and/or iso-nitrogenous EAA control, making it unclear whether the BCAA are truly more anabolic than other EAA in human muscle. The ingestion of high-quality dietary protein providing the full complement of EAA (including the BCAA) more effectively supports the postprandial stimulation of MPS rates than intake of only the BCAA and is required to optimize the acute anabolic response to exercise and nutrition.
Supplementary material
To view supplementary material for this article, please visit https://doi.org/10.1017/S0954422423000197
Acknowledgements
None to report.
Financial support
T.A.C.-V. is supported by a William Dawson Scholar Award at McGill University.
Competing interest
M.S.K., S.J.H., Z.W.B., T.A.C.-V.: none.
Authorship
M.S.K. and T.A.C.-V. conceived the manuscript; M.S.K., S.J.H., Z.W.B. and T.A.C.-V. drafted the manuscript; S.J.H. prepared figures; S.J.H., Z.W.B. and T.A.C.-V. edited and revised manuscript; M.S.K., S.J.H., Z.W.B. and T.A.C.-V. take primary responsibility for the final content. All authors read and approved the final manuscript.