Introduction
Construction industries account for 40% of global greenhouse gas (GHG) emissions and four billion tons of annual waste. Concrete and cement production, responsible for 8% of all industrial GHG emissions, are among the most recorded heavy wastes – accounting for 15–28% of all solid waste (Hu et al., Reference Hu, Kong, Cai, Li, Guo, Su, Liu and Zheng2021; Zhang et al., Reference Zhang, Hu, van der Meide, Di Maio, Yang, Gao, Li, Zhao and Li2023). As such, within the architecture, engineering and construction (AEC) industry, a fundamental shift is required for addressing these negative impacts, with an emphasis on reducing concrete mining, GHG emissions and waste generation, at both material and production workflow levels (Yu et al., Reference Yu, Gu and Ostwald2022). One promising approach that addresses such urgencies is biodesign, a field that proposes integrating processes of living organisms into design workflows, where materials inherit new biological properties (such as carbon fixation and lower ecological footprint), in addition to decreasing the amount of energy used (Myers, Reference Myers2012; Achal and Mukherjee, Reference Achal and Mukherjee2015; Dade-Robertson, Reference Dade-Robertson2020; Qiu et al., Reference Qiu, Artier, Cook, Srubar, Cameron and Hubler2021). While such properties are theoretically desirable, actually integrating biological capabilities in construction materials and building applications is challenging; doing so requires developing an interdisciplinary, multi-scalar approach between architecture and microbiology. One possible facilitator when addressing these challenges relates to newly emerging manufacturing techniques from both disciplines that enhance customization of material properties and production workflows. Manufacturing processes such as additive manufacturing (AM) have demonstrated advantages for sustainability, through efficient resource consumption, reduced energy consumption and costs, decreased pollution and wasteful processes and shortened supply chains (Mehrpouya et al., Reference Mehrpouya, Vosooghnia, Dehghanghadikolaei and Fotovvati2021). Today, using AM workflows, designers are able to interact with new materials, customize printing tool paths for complex material distribution, and enhance the performance of manufactured designs (Mehrpouya et al., Reference Mehrpouya, Vosooghnia, Dehghanghadikolaei and Fotovvati2021; Cohen and Barath, Reference Cohen and Barath2023). Moreover, computer-aided design (CAD) techniques for 3D printing enable the optimization of structural performance and material use (Breseghello et al., Reference Breseghello, Sanin and Naboni2021; Armaly et al., Reference Armaly, Lubov, Shay, Kashi and Barath2023a). In the field of biology, AM in the form of bioprinting has been introduced, to enable high-resolution printing of living materials in spatially defined structures (Balasubramanian et al., Reference Balasubramanian, Yu, Meyer, Karana and Aubin-Tam2021). It would therefore be beneficial to explore the potential integration of AM with living materials for architectural applications, developed through customized biofabrication setups, in order to properly maintain the living organisms (Lim and Thomsen, Reference Lim and Thomsen2021; Thomsen et al., Reference Thomsen, Tamke, Mosse, Sieder-Semlitsch, Bradshaw, Buchwald and Mosshammer2022). Various bacterial activities have drawn the attention of researchers and designers in the architectural discipline, such as bacillus pasteurii’s biomineralization, vibrio fischeri luminescence abilities and cyanobacteria calcium carbonate (CaCO3) production (Metwally et al., Reference Metwally, Mahdy and El-Raheem2020; Qiu et al., Reference Qiu, Artier, Cook, Srubar, Cameron and Hubler2021; Thomsen et al., Reference Thomsen, Tamke, Mosse, Sieder-Semlitsch, Bradshaw, Buchwald and Mosshammer2022). Cyanobacteria is a carbon dioxide (CO2) fixating phylum – one that enhances soil stability in natural habitats, through the biocementation of particles (Dhawi, Reference Dhawi2023). By harvesting solar energy to perform photosynthesis, cyanobacteria precipitate CaCO3, creating a cementitious medium that strengthens bonds between sand particles (Dhawi, Reference Dhawi2023). The effect of cyanobacteria microbially-induced CaCO3 precipitation (MICP) on sand has been utilized in casting processes, for developing self-growing building materials and solidifying sand-based blocks (Qiu et al., Reference Qiu, Artier, Cook, Srubar, Cameron and Hubler2021). Cyanobacteria has also been reported to increase the compressive strength of sand mortar by 25.54%, thanks to MICP (Sidhu et al., Reference Sidhu, Goyal and Reddy2022). Cyanobacterial MICP has also been used in the 3D bioprinting of sand, increasing the compressive strength of the printed structure (Reinhardt et al., Reference Reinhardt, Ihmann, Ahlhelm and Gelinsky2023). MICP within AEC proposes an eco-efficient and less costly alternative to existing concrete and cement production processes. In this study, we strive to replace cement and concrete production – as a means for reducing GHG emissions and waste production within AEC; to do so, we expand on the precedents presented above, by developing the initial steps of a systematic manufacturing workflow for architectural purposes. This workflow utilizes the biological abilities and production of cyanobacteria, for achieving solidification of 3D-printed architectural components, that are sand based, carbon efficient and modular. To the best of our knowledge, cyanobacteria have yet to be printed within an AM biomixture at the architectural component scale. In previous experiments, in our strive to enable 3D printing, we successfully developed a biomixture of cyanobacteria cells, agar and quartz sand, that enabled cyanobacterial growth and MICP (Armaly et al., Reference Armaly, Shay, Kashi and Barath2023b). In this paper, we attempt to overcome biological, mechanical and architectural challenges, in the strive, to develop an applicable AM framework – one that encourages cyanobacterial activity within the printing biomixture. From a biological perspective, factors that must be addressed include bacterial generation time, growth patterns, environmental conditions (such as light exposure and temperature) and metabolite production (Goidea et al., Reference Goidea, Floudas and Andréen2022). From a mechanical perspective, such factors include shear stress, friction and printing methods in relation to cell viability (Persaud et al., Reference Persaud, Maus, Strait and Zhu2022). From an architectural perspective, factors such as strength, stability, appearance, responsiveness and lifespan are crucial (Goidea et al., Reference Goidea, Floudas and Andréen2022). Biological protocols, such as optical density and fluorescence measurements, were applied to two bacterial strains: Synechococcus sp. PCC 7002 and Synechocystis sp. PCC 6803, as a means for encouraging biological activity for AM workflows and enhancing solidification. Assessments of the mechanical delivery system and large-scale printing properties were conducted to customize a suitable robotic deposition system for sand-based inoculated biomixtures. By developing both biological and architectural protocols, we outlined the tradeoffs towards creating a co-fabrication process that enables biological deposition (i.e. the cyanobacterial biocementation through MICP), as well as the robotic deposition of a sand-based biomixture – all within an architectural biofabrication workflow. Harnessing the cyanobacteria deposition as input in the manufacturing workflow, we attempted to bridge the gap between biological and architectural processes, to produce cyanobacteria-based modular architectural components. Not only will such components replace processes that emit GHG, but they will also perform efficient de-facto CO2 fixation throughout the production process.
Designing a co-fabricating workflow
Adopting a design approach that works at more than one scale (in this case, biological and architectural) requires the reexamining of existing processes in each field. Moreover, integrating a living system into the manufacturing process requires the identification of optimal conditions for cyanobacteria growth and MICP, while developing a suitable production workflow. Similar to fabrication processes with living materials (Lim and Thomsen, Reference Lim and Thomsen2021), the co-fabrication workflow should include pre-fabrication, fabrication and post-fabrication phases (Fig. 1), to guide architects in designs that entail cyanobacteria (Armaly et al., Reference Armaly, Shay, Kashi and Barath2023b). To increase CaCO3 deposition and maximize CO2 fixation, we propose a shift, from casting and bioprinting to robotic deposition, leveraging CAD tools at the architectural scale. Doing so could optimize geometrical porosity, increase light exposure and consequently, encourage cyanobacterial deposition within the biomixture (Armaly et al., Reference Armaly, Lubov, Shay, Kashi and Barath2023a). Optimizing geometrical properties for enhancing performance is at the core of architectural CAD techniques and is linked to manufacturing through customized computer-aided manufacturing workflows (Armaly et al., Reference Armaly, Lubov, Shay, Kashi and Barath2023a). In this study, the unique interrelations between biological activities, geometrical properties and robotic AM techniques were leveraged, to generate connections between the biological and architectural scales, while outlining tradeoffs that need to be addressed throughout the three phases of the co-fabrication workflow.

Figure 1. Co-fabrication workflow, with living cyanobacterial cells structured into three phases: (1) Pre-fabrication phase, demonstrating material protocols for culturing cyanobacteria, preparing biomixtures, distributing to cartridges and designing printing tool path and geometrical properties for optimal bacterial activity. The material preparation workflow includes the culturing of cyanobacteria starter, seeding cyanobacterial cells within the biomixture of agar medium and sterilized sand, and incubation of the biomixture culture within suitable environmental conditions – to enable optimal bacterial growth and activity within the biomixture; (2) Fabrication phase, demonstrating the link between design features and material deposition in relation to geometrical, mechanical and environmental parameters; and (3) Post-fabrication phase, demonstrating the appliance of maintenance protocols as a means for prolonging biological activity within the biomixture and increasing solidification.
Co-fabrication workflow phases
First, the pre-fabrication phase includes the developed material protocols for maintaining the cyanobacteria and preparing the biomixture for printing. These protocols range from basic cell culturing (such as cell transfer or seeding) to cell acclimation and growth parameters within new habitats (Armaly et al., Reference Armaly, Shay, Kashi and Barath2023b). This phase also focuses on the effect of the design features on the activity of the living cells, while referring to the effect of design through geometrical, environmental and biomixture printing requirements. For example, the geometrical design (i.e., geometrical porosity and increased surface area) could be informed by environmental conditions, such as increased light exposure, which is necessary for cyanobacterial viability and could enhance biological activity (Clark et al., Reference Clark, McGinley, Purdy, Korosh, Reed, Root and Pfleger2018). Similar tradeoffs regarding geometrical properties and cell behavior include the design of the printing tool path, one that considers cyanobacterial mobility, for example, since sharp angles could reduce uniform integration of the cells with the material (Arellano-Caicedo et al., Reference Arellano-Caicedo, Ohlsson, Bengtsson, Beech and Hammer2021; Armaly et al., Reference Armaly, Lubov, Shay, Kashi and Barath2023a). When fabricating with photosynthetic bacteria, such tradeoffs within AM processes could govern the bacterial viability and in turn, the biological deposition and solidification outcome. Next, the fabrication phase correlates between the effect of the cell viability needs, mechanical setups and printing parameters, such as printing head customization, cartridge size, printing speed and pressure and layer height and width. Potential tradeoffs could relate tool path definitions to shape fidelity, or light exposure to layer width and height; in turn, this could affect cell viability and biological activity. Finally, the post-fabrication phase simultaneously embodies the link between biological activity at the micro and macro scales, as the components demonstrate structural stability, as per the bacterial CaCO3 deposition within the biomixture. The aim of this final phase is to prolong the viability of the cyanobacteria cells, as a means for maximizing the biological deposition within the sand-based biomixture; in turn, this should increase solidification by binding the sand particles together. In this phase, we therefore also apply procedures for maintaining habitat parameters (i.e., nutrition or water) and ensuring suitable environmental conditions (i.e., incubation).
Additive manufacturing constraints on fabricating with living cells
As the utilization of AM in architecture continues to expand, and new material processes are introduced into the AEC industry, biofabrication methods are emerging within the discipline of biology. Bioprinting techniques, for example, can be used to address living material properties and the spatial organization of cells, within regulated environmental conditions that have been customized as the habitat environment of living cells (Persaud et al., Reference Persaud, Maus, Strait and Zhu2022). Unlike traditional construction materials, bacteria cultures are grown under supervised laboratory conditions, while monitoring cell viability and preventing contamination. Certain environmental conditions (such as temperature, light, sterility and nutrition) must be consistently maintained, to ensure optimal bacterial growth. Implementing living cells in a fabrication process differs greatly from controlled laboratory conditions. As such, it is crucial to assess how the environmental conditions of the fabrication setup (such as the temperature of the printing head or of the cartridge) influence the cells. To ensure a balance between the required fabrication setup and the biological conditions needed for maintaining cell viability, certain features must be created through the interdependence of certain mechanical and biological properties. One main mechanical challenge in the current research is maintaining cell viability during and after printing the sand-based construction materials. In construction, pneumatic and screw-driven printing are commonly applied methods for printing aggregate-based materials such as sand (Gomaa et al., Reference Gomaa, Jabi, Reyes and Soebarto2021; Barnes et al., Reference Barnes, Kirssin, Needham, Baharlou, Carr and Ma2022). However, when printing living materials, the mechanical pressure in screw-driven printing could negatively impact cell viability. In biofabrication, pneumatic printing is common in bioprinting procedures as it generates lower pressure forces on cells (Persaud et al., Reference Persaud, Maus, Strait and Zhu2022). While the implementation of pneumatic bioprinting requires further studies for construction materials, it remains promising for live-cell printing (Persaud et al., Reference Persaud, Maus, Strait and Zhu2022). For example, implementing cells within biomaterials (such as agar, gelatin and alginate) has been found to provide a safe habitat from external forces throughout the fabrication process (González et al., Reference González, Mukhitov and Voigt2020). While this could maintain cell viability in a bioprinting setup, the rheological properties of the biomaterials are sensitive and require adaptation within an architectural AM process. As such, essential protocols, such as cross-linking the hydrogel using light, chemical, or thermal procedures (Persaud et al., Reference Persaud, Maus, Strait and Zhu2022), must be introduced into the architectural process – in order to maintain the 3D structures.
Biological and architectural deposition mechanisms
To implement the co-fabrication process and tackle the challenges outlined above, we developed and evaluated a systemic approach that included protocols regarding deposition mechanisms and biological performance optimization. From a biological aspect, the biomixture serves as a safe microenvironment for the living cells; as they are able to gain nutrition and motility through this medium, these cells are also able to perform biological activities within the biomixture, during and following the fabrication process. The medium in which the cyanobacteria are cultured should therefore be biocompatible with their growth, enable the encapsulation of sand particles and be suitable for 3D printing. Agar was chosen as the preferred candidate for our research purposes, owing to its porous structure, biocompatibility with cyanobacteria and ease of nutrition, oxygen and waste exchange (Salati et al., Reference Salati, Khazai, Tahmuri, Samadi, Taghizadeh, Taghizadeh, Zarrintaj, Ramsey, Habibzadeh, Seidi, Saeb and Mozafari2020). Moreover, the ability to adjust the rheological properties of the medium, and its gelation time, could benefit the fabrication process. Yet to correlate between the printing mixture and the bacterial activity, it was important to first understand the cyanobacteria performance, exponential growth phase and optimal concentrations for growth within the mixture (Beal et al., Reference Beal, Farny, Haddock-Angelli, Selvarajah, Baldwin, Buckley-Taylor and Gershater2020). Determining a range of factors, such as cell density and ideal conditions for enhanced colony formation, could be helpful in protocol development processes. At the microscale, this could assist in achieving calculated optimization of the biological deposition within the biomixture, while at the macro scale, this could consequently affect the solidification outcome. Architecturally, the biomixture serves as a sand-based medium for robotic deposition. Printing with sand-based mixtures requires studying the rheological behavior of the mixture, in order to maintain structural integrity (Gomaa et al., Reference Gomaa, Jabi, Reyes and Soebarto2021). In 3D printing processes of sand-based materials, friction poses a major challenge, as excess pressure on mechanical parts could affect both printability (Gomaa et al., Reference Gomaa, Jabi, Reyes and Soebarto2021) and cell viability (Persaud et al., Reference Persaud, Maus, Strait and Zhu2022). To prevent friction and enable the printing of soils (such as cob) and active sand-based materials, the rheological properties of the mixtures can be altered, through water content, for example (Gomaa et al., Reference Gomaa, Jabi, Reyes and Soebarto2021; Barnes et al., Reference Barnes, Kirssin, Needham, Baharlou, Carr and Ma2022). Yet it should be noted that when fabricating with living bacteria, such deviations in material ratios could impact cell viability and biological activity; as such, clear material protocols must be developed, to ensure positive and desirable outcomes. To understand the constraints and thresholds of vital parameters, regarding biological and architectural deposition, we conducted the following experiments in parallel: (1) Biological protocols were applied for identifying parameters that optimize cyanobacterial growth and biological deposition within sand-based mixtures; and (2) Architectural protocols were developed for the robotic deposition of sand-based mixtures. The examined factors include bacterial strain adaptation, mixture material ratios, solidification time, material storage, material delivery and printing head. It is important to note that the applied protocols regarding biological and architectural parameters must be correlated – to ensure cyanobacterial performance within the developed co-fabrication setup.
Studying the CaCO3 deposition within the biomixture
To adapt the fabrication process to the viability needs of the bacterial cells, and enhance biological performance, basic microbiological protocols were applied; this enabled us to further understand the behavior of cyanobacteria – in both laboratory growth conditions and within the biomixture. The main cyanobacterial performance that we aimed at optimizing was MICP through photosynthesis. Cyanobacteria perform photosynthesis through light capturing via chlorophyl, followed by the fixation and conversion of CO2 to CaCO3 (Mehdizadeh Allaf and Peerhossaini, Reference Mehdizadeh Allaf and Peerhossaini2022). Since cyanobacteria growth depends on light absorption through photosynthetic pigments (such as chlorophyl), their growth rate is highly sensitive to light exposure (Campbell et al., Reference Campbell, Hurry, Clarke, Gustafsson and Öquist1998; Clark et al., Reference Clark, McGinley, Purdy, Korosh, Reed, Root and Pfleger2018; Haeder, Reference Haeder2022). In previous research, we developed maintenance protocols for cyanobacterial growth using two strains: Synechococcus sp. PCC 7002 and Synechocystis sp. PCC 6803; this was performed in an incubator that was highly compatible with photosynthetic cyanobacteria growth, as it contained four adjustable fluorescent lights and enabled air circulation. Both strains were grown in a liquid nutrient media (BG-11) and at a constant temperature of
$22\pm 1{}^{\circ }$
C (Armaly et al., Reference Armaly, Shay, Kashi and Barath2023b). Following the success of the initial protocols for establishing bacterial growth within the biomixture (Fig. 2) (Armaly et al., Reference Armaly, Shay, Kashi and Barath2023b), we continued to determine the cyanobacterial growth curve in laboratory conditions, with the aim of enhancing biological activity within the biomixture. This is in line with commonly-used methods for assessing and examining the growth of microbial cultures in laboratory settings, where suitable and consistent nutritional mediums and environmental conditions can be maintained. Once an optimal growth protocol is achieved, a growth curve can then be obtained, by measuring cell numbers, fluorescence, or biomass as a function of time (Maier and Pepper, Reference Maier and Pepper2015).

Figure 2. Cyanobacterial growth within sand agar mixtures. (A. Lefthand image) Initial biomixture experiments of sand casting and deposition. The samples include different biomixtures consisting of two types of quartz sand, thin sand, agar and both bacterial strains; (B. Lefthand circle) Day 0 of sample preparation of sand agar mixture containing cyanobacterial cells; (C. Middle circle) Cyanobacterial growth within the biomixture after 1 week of incubation at a temperature of 22 ± 1∘C; (D. Right circle) Cyanobacteria demonstrating solidification of the biomixture through binding the sand as a united surface (Armaly et al., Reference Armaly, Shay, Kashi and Barath2023b).
Studying a robotic deposition strategy for printing sand-based biomixtures
Within AEC production, the potential of a cyanobacteria-integrated biomixture, for architectural additive fabrication workflows, has yet to be fully explored, especially while utilizing robotic deposition. To correctly bridge AM gaps between architectural and biological procedures, it is important to draw on existing workflows that entail earth-based or living materials. Precedents of printing earth-based materials, with pneumatic and electromechanical extruders (Gomaa et al., Reference Gomaa, Jabi, Reyes and Soebarto2021; Barnes et al., Reference Barnes, Kirssin, Needham, Baharlou, Carr and Ma2022), articulate interrelations between the behaviors and printability of the material. Such constraints must also be addressed in relation to rheological properties and mechanical requirements, as such interrelations impact the viscosity, hardening time and chosen storage and delivery system of the material. In biofabrication, some bioplotters utilize multiple syringes, as cartridges for 3D printing hydrogels. This setup enables the controlling of the printing head temperature, which could impact the rheological properties of the material. It also defines the required material quantities, while enabling printing within sterile and regulated environmental conditions (Ginestra et al., Reference Ginestra, Rovetta, Fiorentino and Ceretti2020). In order to establish protocols for printing cyanobacteria biomixtures, we conducted pneumatic printing tests using a bioplotter (EnvisionTEC 3D-Bioplotter) and a robotic arm (UR5e).
Materials and methods
Material
Cyanobacteria cultures protocol
Two cyanobacterial strains were studied in this research: Synechococcus sp. PCC 7002 and Synechocystis sp. PCC 6803. Both strains were grown in liquid nutrient media BG-11 at a temperature of
$22\pm 1{}^{\circ }$
C, within a Bio-chemical Oxygen Demand incubator with fluorescent lights. The cyanobacteria were weekly re-cultured within a fresh nutritional solution.
Agar medium protocol
The Agar Bacto was prepared in a concentration of 1% (1 g agar: 100 mL deionized water). The medium was stirred on a hot magnetic plate for almost 20 minutes at a temperature of 100∘C. Once a homogenous liquid was achieved, the agar was left to cool down to the required temperature of 25–45∘C.
Sand-based mixtures protocol
Experiments were conducted with various sand agar ratios, such as 1:1, 1.5:1 and 2:1. While the agar medium was still hot and in a liquid state (
$45\pm 1{}^{\circ }$
C), quartz sand, with particles ranging from 0.6–0.84 mm, was added to the agar medium, and manually stirred while the mixture cooled down. The materials were prepared at least half a day in advance, to ensure full gelation and hardening at a room temperature of 25∘C.
Biomixture protocol
The biomixture was prepared using the same protocol as the agar medium while ensuring strict environmental conditions (i.e., nutritional solution BG-11, sterility, light exposure and temperature). As cyanobacterial cells could be harmed by high temperatures, the medium was maintained in a sterile environment as it cooled down. While the agar was still warm (25–45
$\pm$
1°C) and in a liquid state, the cyanobacteria cultures were added, and stirred in gently in a circular motion, to ensure homogenous cell distribution. Prior to gelation, the predefined amount of quartz sand was added and manually mixed into the cultured medium.
Methods
Monitoring cyanobacterial growth and viability
Growth curves were obtained by measuring cell concentration colony forming units (CFU) and auto-fluorescence. Growth and cell viability were assessed based on chlorophyl fluorescence levels. In line with previous studies, the chlorophyl fluorescence was evaluated through spectra analysis of chlorophyl a (max wavelength 650 nm) and chlorophyl b (max wavelength 670 nm), with excitation at 440 nm (Pedrós et al., Reference Pedrós, Moya, Goulas and Jacquemoud2008).
Cyanobacteria growth in cultures
Three parameters were examined during the experiments: (1) optical cell density (OD); (2) CFU; and (3) fluorescence as a function of time for optimizing biological deposition. We examined the growth of the starter cultures every 2 days, over a 2-week period. To examine relative cell density for each strain, 600 nm OD measurements were conducted. We also examined an absorption spectrum of wavelengths, ranging from 600 to 740 nm. Fluorescence measurements were applied to the same cultures, to examine the acclimation status and photosynthesis activity of the cyanobacteria, using an epi-fluorescent microscope Axiolab 40 (Carl Zeiss, Germany) that enabled visualization of bacterial fluorescence via a green filter of 520 nm.
Cyanobacteria growth in biomixtures
The biological viability of the two selected strains of cyanobacteria was tested in biomixtures in different sand-agar ratios as per the co-fabrication process. The cell viability in the biomixture samples was assessed by fluorescence levels and microscopy visualization of the chlorophyl (a and b). The fluorescence levels of the biomixture samples, which comprise a multi-component system, are strongly influenced by the composition of the sample, which may include fluorescent components. For purposes of this study, a negative control was created through mixtures of sand and agar without cyanobacteria, while a bacterial inoculum of the same concentration was used as a positive control.
Bioprinting
The aim of the bioprinting experiments that we conducted was to examine the printing of a sand-based biomixture within a biologically-adapted setup. Such settings prioritize cell viability, as they enable full control of environmental conditions, from regulating the temperature of the printing head to enabling the placing of the entire setup within a biological hood, thereby ensuring a sterile setting for both the materials and the printing environment. As the medium properties may affect cell viability, it was important to examine its printability and the printability of its integration with sand. In the prefabrication phase, a grid geometry was designed, using Rhinoceros 3D, and then inserted into Perfactory RP and VisualMachines software. Throughout the fabrication phase, printing parameters such as printing head temperature and pressure were tested in the following ranges: printing head temperatures, 15–45∘C; printing speeds, 1–65 mm/second; pressure, 0.1–1 bar; and printing nozzle diameters, 1.1–2.6 mm.
Robotic printing
The fabrication workflow was tested on the sand-based mixtures while considering both printability requirements and cyanobacterial needs. The defined sand agar ratios were determined, to enable the examination of the material viscosity impact on printability. Moreover, to correctly assess the printability of the various ratios, a single-layered tool path was designed using Rhinoceros 3D, Grasshopper and HAL Robotics frameworks – to enable parametric adjustments of geometric variables. Next, multi-layered components were printed, to enable the examination of material adhesion and shape fidelity. In the fabrication phase, two nozzle types were tested regarding the material’s printability – conical and cylindrical – to tackle material clogging. When governing the printability of the mixtures, material viscosity, aggregate size and environmental temperature were assessed. First, the experiments were manually extruded to examine deposition feasibility, and only then were they automated, utilizing air pressure and a customized printing head.
Results and discussion
Cyanobacterial growth in culture
The cyanobacteria were grown under laboratory conditions, in order to incorporate them into the biomixtures; their growth was monitored based on auto-fluorescence, cell viability (CFU of the exponential growth phase) and OD measurements. Based on the preliminary results, the following estimations were applied: after inoculation of fresh cultures (where visual pigmentation is still absent), the exponential growth phase of Synechocystis sp. PCC 6803 was estimated to begin on day 7, reaching a concentration of 5.7 × 107 CFU/ml. The exponential growth phase of Synechococcus sp. PCC 7002 was estimated to begin on day 9, reaching a concentration of 5.0 × 107 CFU/ml (Fig. 3). The starting timepoint of the exponential growth phase was defined for each strain, serving as a base for identifying the optimal concentration of cells that should be added to the biomixtures. Doing so could potentially help time the co-fabrication process while constructing a workflow that is synchronized with the biological activity.

Figure 3. Representative bacterial growth curves for Synechocystis sp. PCC 6803 and Synechococcus sp. PCC 7002 at a wavelength of 600 nm OD. An average of two replicates is presented. The image of the developed biomass in the tube is given for each time point.
Biomixture
The biological viability of the two selected strains of cyanobacteria was tested in biomixtures in different sand-agar ratios, to optimize the co-fabrication process. The cell viability in the biomixtures samples was assessed by chlorophyl fluorescence level (Fig. 4). The preliminary results suggest that the fluorescence of the biomixture in the sand and agar mixtures increases with time. This indicates that cyanobacteria were able to survive and thrive within irregular environmental habitats, in this case – the sand.

Figure 4. Representative biological viability tests of cyanobacteria cells (Synechococcus sp. PCC 7002 and Synechocystis sp. PCC 6803) that were first grown under laboratory conditions and then added into the biomixtures of 1.5:1 sand agar ratio over a 4-day period. The cell viability in the biomixtures samples was assessed by chlorophyl fluorescence level (440 nm ex/650 nm em). The measured fluorescence was normalized with the background fluorescence of the mixture, without the cyanobacteria.
Bioprinting
The medium demonstrated continuous printing at the following settings: (1) low room temperature, 15–25∘C; (2) low printing speed, maximum 10; (3) pressure >1 bar (0.1–0.5); and (4) nozzle diameter, 2.1 mm. The printing setup was successful in the 3D structuring of the agarose habitat medium, yet it was unsuccessful in printing the sand-based mixtures at the developed biomixture ratios. The difference between the syringe body and narrow tip diameters led to accumulation of sand particles at the nozzle opening, which in turn led to clogging and increased friction between the sand particles. Although the printing was not found to be suitable for the sand-based mixtures, we adopted the syringe as a cartridge bioprinting setup for the robotic printing experiments at the architectural scale. This was done in order to avoid material contamination in non-sterile environments (Thomsen et al., Reference Thomsen, Tamke, Mosse, Sieder-Semlitsch, Bradshaw, Buchwald and Mosshammer2022). An upscaled cartridge delivery system has been designed by Gomaa et al. (Reference Gomaa, Jabi, Reyes and Soebarto2021) for earth-based construction materials within a robotic fabrication workflow. Although this setup does not involve living materials, it tackles relevant obstacles, such as material storage, delivery and printing consistency – all of which are vital for a successful co-fabrication workflow within this research and serve as a base for our developed fabrication setup.
Robotic printing
The robotic printing experiments conducted in this study enabled the identification of bioprinting setup advantages, including the printing head cartridge and the regulated environmental conditions. Doing so enabled the development of an upscaled printing workflow with a UR5e robotic arm – one that is suitable for sand-based mixtures with cyanobacteria (Fig. 5). When testing the conical nozzle, all experiments resulted in material clogging, at various phases of the printing process, and regardless of the ratios. First, the material deposition remained limited, similar to the bioprinting experiments detailed above. Modifications of the printing head tip enabled alterations to the slope angle and length, which determined the fluidity of the material: longer and sharper angles increased clogging, while shorter and wider angles increased the flow. The alteration of both factors also determined the printing diameter, leading to undesired changes in the printing resolution; On the other hand, cylindrical nozzles enabled successful printing, without any clogging and at all predefined material ratios. These were printed with pressures >1 bar, similar to the bioprinting experiments detailed above. Interestingly, a low sand ratio (1:1) demonstrated better deposition flow, while a higher sand ratio (1:1.5 1:2) demonstrated higher shape fidelity, based on the tool path definition. As higher quantities of sand within the mixture resulted in fractions and inconsistency in the printed layer, a ratio of 1:1 proved to be preferable.

Figure 5. Initial robotic deposition experiments of sand-based mixtures. (A. Left) Single layer robotic deposition of sand-based mixtures in the developed ratios. The examined factors include: material ratios, material delivery system and printing head customization to avoid clogging. (B. Right) Multi-layer robotic deposition of sand-based mixtures in 1:1 sand agar ratio. The multi-layered components examine the correlation between toolpath definition and material shape fidelity.
The initial set of printing experiments presented in this paper enabled us to determine a range of parameters that are critical for defining material viscosity, environmental conditions and mechanical requirements – in relation to both the printing head and the printing method. Utilizing this knowledge, we outlined the following customized printing setup, that is suitable for cyanobacterial activity within sand-based mixtures: (1) cylindrical cartridges are utilized, to decrease material waste, ensure cell viability and prevent increased friction; (2) printing material quantities and cartridge volume are defined and limited by the generation time of the bacteria and their growth speed, which determines the amount of living material available for use. Currently, 60 cm tubes with a 0.98 diameter are being tested as cartridge prototypes. The aim is to eliminate the need for external feeding systems while enhancing maintenance and regulation of the environmental conditions when embedding the cells in the cartridges. (3) Moreover, the proposed setup utilizes multiple cartridges that plug into pneumatic pressure; working sequentially, this enables the unused cartridges that contain the biomixture to be incubated, maintain bacterial growth and avoid contamination (Fig. 6). Such fabrication setups could enable the production of relatively large-scale architectural modular components, such as blocks and panels. We are currently working on the design of architectural components, subject to the size constraints of the incubator (40 × 40 × 40 cm) (Armaly et al., Reference Armaly, Lubov, Shay, Kashi and Barath2023a). When addressing 3D concrete and earth printing within architectural production, tool path designs impact the components’ fabrication process and their structural performance (Breseghello et al., Reference Breseghello, Sanin and Naboni2021; Gomaa et al., Reference Gomaa, Jabi, Reyes and Soebarto2021). It is also important to consider increased surface areas for increasing light exposure when designing tool paths for photosynthetic cyanobacteria. By utilizing CAD tools in the design of these components, such geometrical properties could be further optimized, as per the given lighting conditions within the incubator; in turn, this could encourage cyanobacterial activity, while impacting the solidification of the architectural components (Armaly et al., Reference Armaly, Lubov, Shay, Kashi and Barath2023a). Once the cyanobacteria biomixtures are successfully integrated within the developed fabrication setup, future steps should rely on the accumulated results and conclusions. Doing so will enhance adherence to construction requirements, by optimizing the cyanobacteria biomixture for increased solidification. Moreover, this could adapt the developed fabrication workflow, for customized carbon-efficient modular component production and potentially allow large-scale printing using a cyanobacteria biomixture.
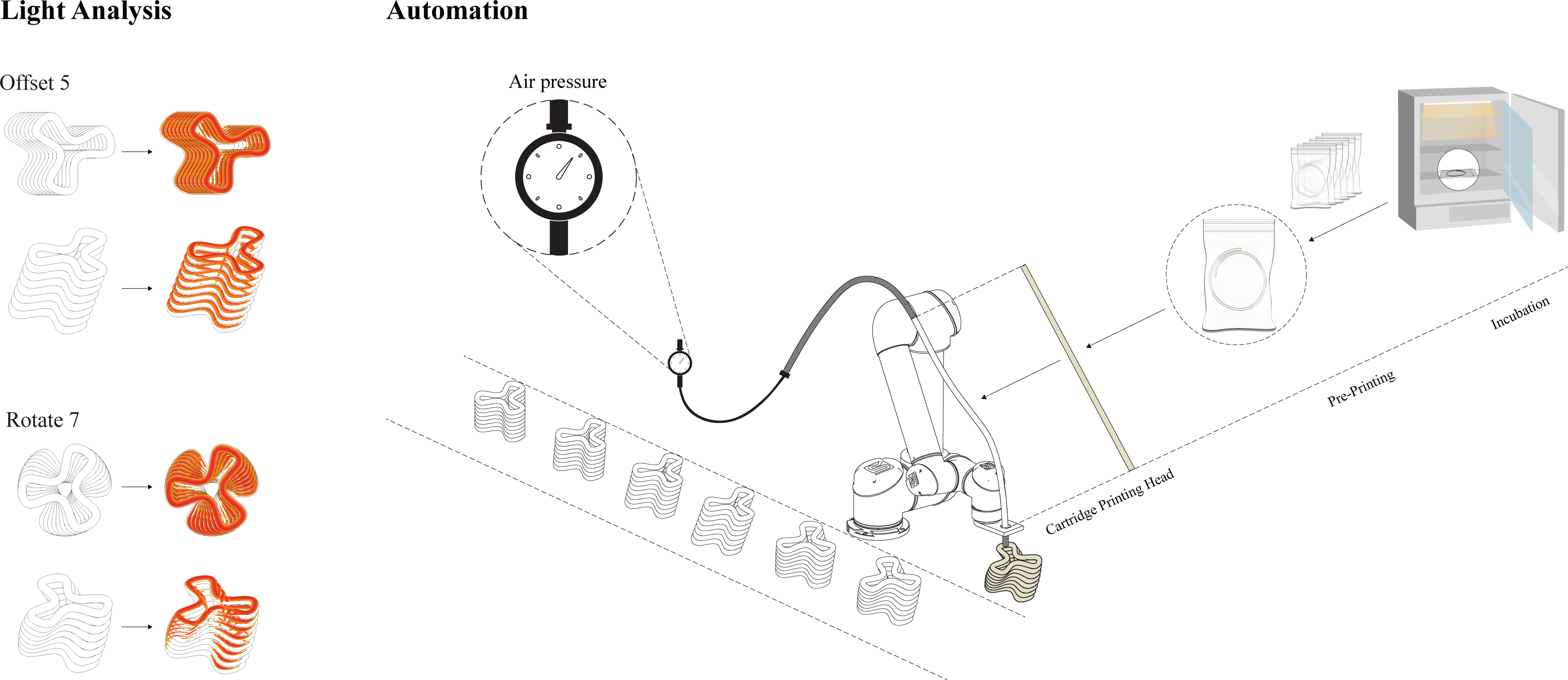
Figure 6. Diagram, (A. Left) Light analysis, Optimized geometries for increased light exposure through increased surface area. The optimization utilizes CAD tools and applies two design principles: offset and rotate, for increasing surface area (Armaly et al., Reference Armaly, Lubov, Shay, Kashi and Barath2023a). (B. Right) Suggested automation workflow for the upscaled biofabrication properties within a robotic AM setup for architectural production (Armaly et al., Reference Armaly, Lubov, Shay, Kashi and Barath2023a).
Conclusion
This paper presents a novel design approach to producing bio-based architectural components, by leveraging AM processes based on biological data regarding cyanobacteria. The experiments and protocols detailed in this study tackled challenges within the co-fabrication process, while constantly examining tradeoffs between biological and architectural requirements. Through advanced biological procedures, two bacterial strains were examined for bacterial deposition. An ideal cell seeding time was defined for each strain, based on OD measurements and fluorescence examinations. In addition, an initial CFU count for cyanobacteria was conducted (and which is currently being optimized in further experiments), to achieve more accurate assessments of culture concentrations. In the future, we plan on examining the presence of CaCO3 in cyanobacterial cells, as well as the amount of precipitated CaCO3 versus the amount of fixed CO2. Future studies could benefit from comparing carbonate quantities in the sand mixture, prior to and following the addition of the cyanobacteria to the biomixture. For further optimization, the previously developed protocols will be reexamined in relation to environmental conditions (temperature and light) and nutrition (medium). By constructing such protocols for optimized biological activity within the biomixture, we aim at correlating the photosynthetic performance and the biological deposition towards the design of light-sensitive geometries. Moreover, we were able to identify links (albeit weak ones) within the material delivery system, as well as adjusted factors that influence printability (such as friction, viscosity and pressure). This was achieved through preliminary printing experiments that utilized a cartridge printing head. In the future, we plan on conducting experiments to examine cell viability in relation to CFU, biomass production and material strength. In addition, the link between geometry and biological activity will be examined on the architectural scale. We hope to achieve this through printing geometries that focus on a range of design resolutions, from tool path, line width and layer thickness to geometry orientation to light, increased surface area and potential structures that enhance solidification. The experiments presented in this study illustrate the important potential of the co-fabrication workflow. Through collaboration between architects and biologists, we aim at improving and encouraging mutualism between building materials and their surroundings, to allow designers to become active participants in fostering sustainable environments.
Data availability statement
The data that support the findings of this study are available from the corresponding author, [PA], upon reasonable request.
Acknowledgement
The authors thank Avraham Cohen and Yoav Dabas from Disrupt. Design Lab for their support in the development of the automation workflow.
Funding Statement
This work is supported by Technion Additive Manufacturing and 3D Printing Center [Grant Agreement ID 86638420].
Competing interests
None.
Comments
No accompanying comment.